Introduction
Collagen belongs to the class of fibrous protein and is the most abundant polymer in animals constituting about 25-30% of protein mass in humans (Cruz et al., 2021). Collagen is classified into 28 subtypes till date and there are 5 most common types present in humans, amongst which, the most prevalent type of collagen is present in skin, cartilage and tendons is Type I (Blum, 2011). Cartilage is made up of Type II collagen, Reticulate fiber is made up of Type III collagen, Extracellular matrix (ECM) has Type IV collagen, Cell surface, hair and placenta are formed of Type V collagen (Sun, Li, Gong, & Feng, 2021). Type 1 is the significant and abundant type of collagen due to its ubiquitous presence in connective tissue fibers (Hulmes, 2002). The chief role of this particular collagen is to provide mechanical and organizational support to the ECM (Vogel, 2001). In the case of diabetes and aging, changes in tissue stiffness are due to the non-enzymatic cross-linking of collagen and with respect to arthritis, the fibrillar collagen confirmation is misidentified as non-self by matrix metalloproteinases mediated proteolysis of cartilage thereby leading to tissue damage (Rosloniec, Cremer, Kang, & Myers, 2001).
Collagen is made up of two α1 chain and α2 chain forming a triple helix structure and the most abundant motif in the sequence of amino is Glycine, Proline and X (where X can be any amino acid other than Glycine, hydroxyproline and Proline) (Shenoy et al., 2022). In the triple helix stand, 18 amino acids are present in one turn also the left-handed helix is formed by the molecule's three α-chains (Hofmann, Fietzek, & Kühn, 1978). The helical structure is created when the three chains that are distanced from one another by one residue in a right-handed manner around a central axis is supercoiled (Fraser, Macrae, & Suzuki, 1979). Although collagen contains hydroxyproline significantly at higher concentrations than the other proteins, this amino acid is not unique to collagen alone and is therefore employed as a particular index of collagen synthesis and concentration (Shoulders & Raines, 2009). As shown in Figure 1 (a and b), there is at least one triple-helical region in every molecule of collagen and confirmation of the same makes the helical structure extremely proteolytic resistant because, the peptide bonding with adjacent amino acids are hidden inside the molecule (Brodsky, Thiagarajan, Madhan, & Kar, 2008).
Collagens are essential for the healthy development and growth of any individual animal and humans as well. Mutations in the various collagen genes are in charge of numerous clinical diseases pertinent to the skeleton, skin, and joints (Campos, Junior, Pimentel, Carregã, & Cazarin, 2023). Collagens are crucial to the healing of wounds, i.e., platelets are activated by fibrous collagen leading to a hemostatic clog formation, granulation of tissue and control of cell migration after injury to various types of tissues (Mathew-Steiner, Roy, & Sen, 2021). Collagen can operate as survival factors and often stop the adhering cells from dying. Other than normal growth and support to body tissues, collagen also is an inhibitor of angiogenesis. Proteolytic fragments of Type XVIII are Endostatin (Marneros & Olsen, 2005), Restin is a proteolytic fragment of type XV collagen (Mutolo et al., 2012) proteolytic fragment of Type IV Collagen such as Arrestin, Canstatin, tumstatin have been found in the extracellular matrix, which are potent endogenous inhibitors of tumorigenesis (Arakaki et al., 2021).
The extracellular matrix of the human skin tissue comprises 3 main constituents like glycosaminoglycan, proteoglycans, and fibrous protein. Of the fibrous proteins, collagen is a building block for skin, bone and cartilage (Frantz, Stewart, & Weaver, 2010). As positive feedback to injury, collagen invites platelet activation and aggregation of WBC (white blood cells) thus leading to fibrin clot in the site of injury. Collagen degradation releases peptide factors responsible for the proliferation of fibroblast and growth factors responsible for re-epithelialization and angiogenesis (Mathew-Steiner et al., 2021). The type and the amount of organization in the collagen determines the elasticity and strength of the recovered wounded tissue. Accumulation of type III collagen at the wounded area marks the initial stages of wound healing, which is further modified into type I collagen (Singh, Rai, & Agrawal, 2023). Lysyl oxidase enzyme enhances the initial accumulation of collagen during the granulation tissue formation, induces covalent bond linking for the final conversion and modification of collagen (Reilly & Lozano, 2021).
Figure 1
Figure 1a. The common motif of collagen– Glycine, Proline, Hydroxyproline. Figure 1b. Triple helix structure of collagen. The side chains of proline and Hydroxyproline (shown in red) are on the outside of the molecules and the bonds (shown in green) are the peptide bonds between the amino acids present inside the triple helix. The hydrogen bonds shown in blue dotted lines stabilize the triple helix.
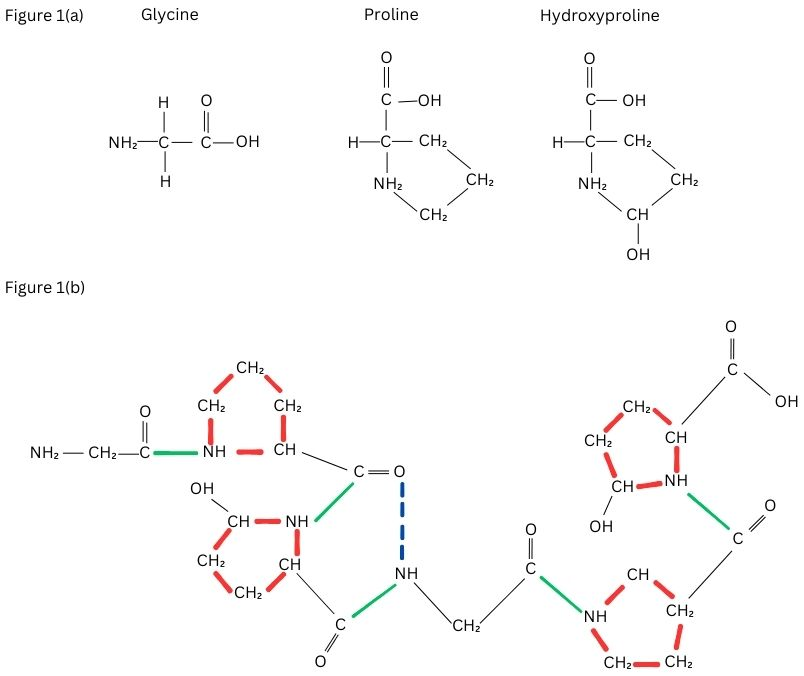
Collagen peptides are formed in the ribosome alongside the endoplasmic reticulum, after mRNA is translated, the formation of α1 and α2 chains begins. These peptide strands are called pre-procollagen and have the capacity of signaling amino acid sequences on their terminal ends (Dunsmore, 2006). The pre-peptides are further moved to the Rough Endoplasmic reticulum and these signaling peptides are broken down in the endoplasmic reticulum leading to the formation of pro-alpha chains. Amino acids such as lysine and proline are hydroxylated in the lumen, and certain hydroxylysine are then further glycosylated. Procollagen is synthesized in the endoplasmic reticulum with 2 strands of α1 chain and 1 strand of α2 chain.
Animals are buildup of macroscopic fibres and networks found in bone, tissue and basement membranes using a single collagen triple helical structure known as tropocollagen, which assembles in a stratified manner. Collagen is widely distributed, making the triple helix structure of recurring N-H(Gly)O=C(Xaa) with hydrogen connections, which are abundant in the animal kingdom i.e., amide-amide hydrogen bond (Jenkins, Vasbinder, Miller, & Raines, 2005). Based on their supramolecular organization and structure, collagens are split up into fibril-forming collagens, fibril-associated collagens, network-forming collagens, anchoring fibrils, basement membrane collagens, transmembrane collagens and other types based on the particular activity (Gelse, Pöschl, & Aigner, 2003). The tropocollagen of type I collagen undergoes random coiling confirmation as the tropocollagen is unstable at body temperature (Leikina, Mertts, Kuznetsova, & Leikin, 2002). Furthermore, collagen must be assembled into strong macromolecular structures in order to support the torsional stress in any given dimension (Buehler, 2006).
Recent information has made it possible to regulate the structure of the collagen cartilage fibrils to an instant resolution (4 nm). The structure demonstrates the heterotypic microfibril structure of collagen fibrils in cartilage, which means that the surface of the fibril has ten evenly spaced microfibrils, while the fibril core has four equally spaced microfibrils (Holmes & Kadler, 2006). Type I collagen fibres in tendons can be up to 1 cm and 500 nm in length and diameter, respectively. The diameter and length of a single triple helical collagen structure are both about 300 nm. Clearly, the natural collagen fibril’s structural dimensions need fibrillogenesis on an exceptional scale. The fact that collagen fibrils are D periodic (D = 67 nm) is their most distinguishing trait. A tropocollagen monomer's accurate length is 4.46D, resulting in 0.54D and 0.46D gaps and overlaps respectively, which causes the banded structure seen in images of collagen fibrils through transmission electron microscopy. The collagen fibril and microfibril structural models must take into account this regular overlap region and an array of gaps (Shoulders & Raines, 2009).
Hydroxylase enzyme is involved in the final modification of collagen from pro-collagen, while in case of disorders like scurvy, deficiency of Vitamin C occurs. This vitamin C acts as a cofactor for the synthesis of Hydroxylase enzyme.
The kind, quantity, and organization of collagen, which alters as the wound heals, determine the tensile strength of the recovered skin. Wound healing involves four major steps: Homeostasis, Inflammation, Proliferation and Maturation/Remodeling (as shown in Figure 2). During the initial phase of wound healing (as mentioned in Figure 2), type III collagen is synthesized, later replaced by type I collagen, one of the prominent skin collagen types (Gould, 2016). The lysyl oxidase enzyme enhances the first random deposition of collagen and promotes covalent cross-linking during the development of granulation tissue (Mathew-Steiner et al., 2021). If the wound heals without any interruptions, collagen remodeling occurs for several months, and the restored tissue gains tensile strength that is around 80–85% of that of the unrestored tissue (Fitridge & Thompson, 2011).
During healing, the major producer of collagen is fibroblast (Sorushanova et al., 2019). The biosynthesis processes of collagen that produce fibrils have been the subject of most investigation and involve a number of complex phases in the spatial and temporal coordination of many biochemical activities (Liu, Zheng, Luo, Wang, & Jiang, 2019). After transcription, the endoplasmic reticulum modifies the nascent/pre-pro-collagen through post-translational modification, removing signal peptides from the N-terminus to create pro-collagen. The triple helical collagen structure is created as a result of the glycosylation and hydroxylation of amino acid (Owczarzy et al., 2020). The triple helical pro-collagen framework is further supported by chaperone proteins and packaged for processing and maturation of the pro-collagen takes place in the Golgi apparatus.
Figure 2
Indicates the stages of wound healing. A), Homeostasis, B), Inflammatory Phase, C) Proliferative Phase, (D) Remodeling Phase.
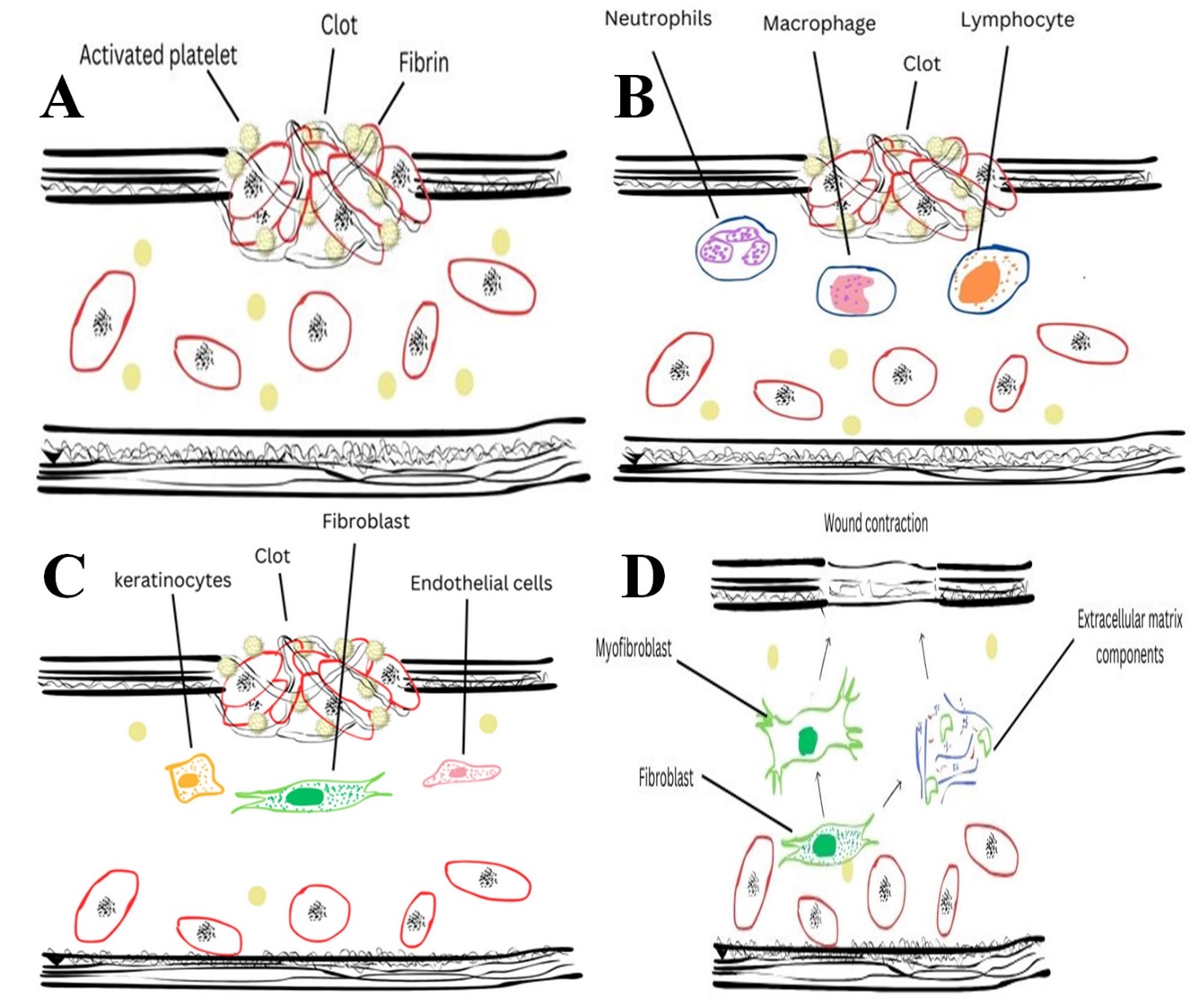
After being put together into secretory vesicles, the pro-collagen is subsequently extruded into the extracellular space, where it undergoes enzymatic modification to become tropocollagen (Revell et al., 2021). The cross-linking process includes disulfide bonds, cross-links (mature) are produced through the lysyl oxidase pathway. Advanced glycation and transglutaminase cross-links, end product-mediated cross-links, define the mechanical property of collagen, i.e., elasticity and reversible deformation. A multi-layered hierarchical structure is created by minute differences in cross-linking that depend on the type of collagen and the uniqueness of the tissue (Liu et al., 2019).
Since the 1990's, after the emergence of the term tissue engineering, in order to contribute to structural stability and a favorable domain for cellular regeneration, scaffolds based on biomaterial have been a study of interest, functionally "imitating" the original tissue (Zhong, Zhang, & Lim, 2010). The selection of an appropriate polymer is an important step in the preparation of scaffolds. Collagen, Hyaluronic acid, chitosan, gelatin and fibrin, and silk are a few examples of biopolymers that have been applied as scaffolds. Scaffolds are made by synthetic or natural biomaterials in various forms such as nanofibers, sponges, 3D structures hydrogen mats, foams, nanogels and membranes (Jafari et al., 2017). Wound healing scaffolds are created using a variety of techniques and a few of them are extrusion, molding, freeze-drying and electrospinning. Availability for use, lower cost, flexibility, and scalability, the electrospinning process continues to be among the most popular of these (Ramalingam et al., 2019).
The following essential components should be present in a scaffold used for wound healing applications: suitable mechanical and physical properties, proliferation, and differentiation of high physiological backdrop to enable cell–to-cell adhesion (Negut, Dorcioman, & Grumezescu, 2020). The scaffold should ideally be both biocompatible and biodegradable, rate of degradation that coincides with the time required for the wound to heal. A moist environment must be maintained by the scaffold in order to offer vital cues for cellular adhesion, migration, and growth to trigger angiogenesis, to accelerate the development of granulation tissue followed by re-epithelialization (Talikowska, Fu, & Lisak, 2019).
Porcine-based and bovine-based collagen application has been forbidden due to religious constraints, dietary regimes and specific needs. Additionally, foot-and-mouth disease, transmissible spongiform encephalopathy and bovine spongiform encephalopathy (Kumagai, Daikai, & Onodera, 2019) that occurred over the past few years around the world, primarily in Asia and the United Kingdom, a wider segment of the population began to express concern about the use of products derived from cows (Coppola et al., 2020). Because they may serve as a means of spreading these diseases, the usage of items generated from cattle is greatly restricted (regulations CE n. 999/2001 and UE n. 142/2011). Therefore, there is a need for an alternative source of collagen.
In this light, the last 20 years there has been a significant increase in interest in marine sources (such as fish, Mollusca and sponges) as secure, prominent and alternative sources for the production of collagen. Marine collagen is free of religious objections and exhibits a naturally decreased threat of communicable infections. Additionally, the fishing industry generates an array of waste or byproducts (such as skin, heads, fins, bones, intestines, and scales) per year that make up roughly 85% of the average catch weight and cause serious damage to the environment (Pal & Suresh, 2016). Fish byproducts are therefore valuable for collagen production, making fish collagen both profitable and cost-effective while still being environmentally beneficial (Liu et al., 2015).
Despite the constructive outcome of fish-based collagen, there is still only a limited understanding of the use of fish collagen into wound healing. The idea of this review article is, to sum up the recent use of fish collagen in preparation of wound healing scaffolds. The extraction of collagen, preparation of scaffold and linkages in preparation of scaffolds, merits and demerits of use of fish collagen are dealt with in detail. In this context, the purpose of the review was to perform a systematic literature survey to explore the effect of fish-based collagen tissue wound healing in experimental models with reference to in-vivo studies.
Review Protocol
Systematic Review Centre for Laboratory Animal Experimentation (SYRCLE) guideline was followed to perform the review analysis. The review was performed during the month of April and May 2023 using Google Scholar and PubMed databases. The literature review was performed according to the directions of PRISMA. The MeSH terms were defined as “Fish Collagen” AND “Wound Healing” AND “Scaffold”. Further, other synonyms and advanced searches were used for detailed understanding.
Eligibility criteria
Data extraction
The important variables examined here are scaffold preparation and wound healing. Moreover, other variables like biomaterial type, animal sex, weight, age, wound size, wound type, implantation time, extraction protocol of collagen, protocol of scaffold preparation, cross-linking method, in-vivo analysis performed and the overall results were analyzed.
Results and Discussion
The PRISMA flow diagram represents the search scheme of the current review (Figure 3). A total of 36 research papers were retrieved from the Google Scholar and PubMed databases. The duplicated articles were removed (n=1) Thirty-five full research articles were evaluated for the inclusion criteria out of which, 25 articles were removed based on exclusion criteria. Eventually, 10 research articles were included and analyzed in the current review.
DTSCS- Dialyzed tilapia skin collagen sponges; STSCS- self-assembled tilapia skin collagensponges;EDC/NHS- N-hydroxysuccinimide,1-ethyl-3-(3-dimethylaminopropyl) carbodiimide hydrochloride; FSC- Fish scale derived collagen
From Table 1, it is evident that collagen was used as the biomaterial and certain studies have extracted collagen from a specified fish scale while few other studies have not specified the species of the fish collagen. Of the 10 studies included in this review, 4 studies have extracted collagen from tilapia (Sun et al., 2021; Wang, Yang, & Wang, 2021; Zhou, Sui, Mo, & Sun, 2017), 1 study extracted collagen from Panaeolus olivaceus (Chandika et al., 2021), 1 study extracted collagen from Cyprinus carpio (Hartinger et al., 2020) 1 study extracted collagen from Ctenopharyngodon idellus (Shi et al., 2020), 1 study extracted collagen from Scyliorhinus canicula (Lahmar et al., 2022) 2 studies have not specified the fish species because both these studies obtained fish collagen from laboratories.
depicts the variables assessed in the included studies, species/strain, age, weight, skin wound size, animal sex, implantation period and protocol of treatment. The animal models used for these are ICR mice (Chandika et al., 2021), Wistar rats (Hartinger et al., 2020) Sprague- Dawley rats (Hu et al., 2021; Sun et al., 2021; Wang et al., 2021; Zhou et al., 2017) New Zealand White rabbits (Shi et al., 2020), BALB/c albino rats (Lahmar et al., 2022) Mice (Li et al., 2018). Five Studies used male animals (Chandika et al., 2021; Hu et al., 2021; Sun et al., 2021; Zhou et al., 2017) remaining five studies have not specified the sex of the animal model. The age of the animal varied from 6-10 weeks (Chandika et al., 2021; Hu et al., 2021; Lahmar et al., 2022; Wang et al., 2021; Zhou et al., 2017). However, the remaining five studies have not reported the age of the animal model. The weight of the animal varied mostly between 200-380g in the nine studies reported except for one study where the weight of the mice reported was 30g (Chandika et al., 2021). The skin wounds were of 3 different types, five studies performed excision wounds (Chandika et al., 2021; Hu et al., 2021; Lahmar et al., 2022; Zhou et al., 2017), three studies performed Incision wounds (Hartinger et al., 2020; Li et al., 2018; Wang et al., 2021) and two studies performed on burn wounds (Shi et al., 2020; Sun et al., 2021). The wound size varied from 0.5 to 2 cm.
Most of the studies used one-time treatment and there was no change of dressing or scaffold. The scaffolds were inserted and sutured (Hartinger et al., 2020; Hou et al., 2020; Li et al., 2018; Shi et al., 2020; Sun et al., 2021; Wang et al., 2021). One study changed the dressing at regular intervals of two days (Lahmar et al., 2022). The remaining three studies used sponges for treatment (Hu et al., 2021; Sun et al., 2021; Zhou et al., 2017). Table 2 shows the details about the extraction protocol of collagen, membrane manufacturing technique, physical and morphological characteristics of scaffolds of the prepared scaffolds and sponges. Of all the extraction protocols used, extraction using acetic acid is the most common technique (Chandika et al., 2021; Hartinger et al., 2020; Lahmar et al., 2022; Li et al., 2018; Shi et al., 2020; Wang et al., 2021). Some of the studies were based on the obtained fish collagen commercially (Hou et al., 2020; Hu et al., 2021; Sun et al., 2021; Zhou et al., 2017). Five studies used N-hydroxysuccinimide (NHS) and 1-ethyl-3-(3-dimethylaminopropyl) carbodiimide hydrochloride as crosslinking agents (Hartinger et al., 2020; Hou et al., 2020; Hu et al., 2021; Sun et al., 2021). Two studies used glutaraldehyde as a cross-linking agent (Shi et al., 2020; Zhou et al., 2017). Three studies have not specified about the cross-linking agents used (Lahmar et al., 2022; Li et al., 2018; Wang et al., 2021).
Table 1
List of studies using collagen from marine sources, the types of animal model used, the type of woundcreated and protocol of wound dressing performed on animal models
Table 2
Presents the collagen extraction protocol, the crosslinkers used, and scaffold preparation technique.
[i] PCL- Polycaprolactone;DTSCS- Dialyzed tilapia skin collagen sponges; STSCS- self-assembled tilapia skin collagen sponges; Col- Collagen; PSC- Pepsin soluble collagen; FSC- Fish scale derived collagen; PLGA- Poly (lactic-co-glycolic acid); FC- Fish collagen; ASC- Acid soluble collagen; Col/NAC: collagen (Col) with N-acetylcysteine
Table 3
Presents the basic parameters of scaffolds quality
Table 4
Overall results of wound healing on different animal models
Freeze drying technique was the most common type of technique performed in the preparation of collagen-based scaffolds or sponges $. Four studies used the electro-spinning technique for the preparation of scaffold (Chandika et al., 2021; Hu et al., 2021; Sun et al., 2021; Zhou et al., 2017).
Pore size is one of the important parameters in scaffold preparation as these pores act as a niche for the developing cells and the higher the pore size greater the growth of the developing cells, similarly this can be achieved by the addition of collagen at a higher ratio. From the above studies, it is also clearly evident that collagen alone cannot be used for the preparation of scaffolds due to its lower melting point and tensile strength. Hence, most of the studies involve the synthetic polymer and crosslinking agents to stabilize the structure. Other parameters which determine the quality of scaffolds are biodegradability, tensile strength and water absorption capacity. Table 3 represents the details of the scaffold’s pore size and of which one study didn’t examine the mechanical or structural properties of the prepared scaffold (Lahmar et al., 2022). One study showed the highest pore size (Shi et al., 2020). Table 4 represents the wound healing rate in animal models and also its histological analysis. Of all the studies, one study showed a complete wound healing on day 21 (Wang et al., 2021) while all other studies showed a longer time duration (up to 30 days) for a complete wound healing. The cellular behavior in the case of wound healing is directly affected by the scaffold architecture as ECM influences specific Integrin-ligand interaction between the cells and its surrounding environment (Murphy, Haugh, & Brien, 2010). All subsequent actions inside the scaffold such as proliferation, migration, and differentiation are mediated by the initial cell attachment (Anselme, 2000). Because the cells are able to recognize small ECM changes that may have an impact on their behavior, pore size can have an impact on a number of biological scaffolding parameters, including cell attachment, infiltration, and vascularization (Boyan, Hummert, Dean, & Schwartz, 1996; Harley et al., 2008). As a result, a balance must be maintained between the proper pore size for cell movement and a specific surface area for cell adhesion (Karageorgiou & Kaplan, 2005).
Table 5
Presents the different techniques used for the fabrication of scaffolds along with their advantages and disadvantages
Technique | Advantages | Disadvantages |
Freeze Drying (Fereshteh, 2018) | No involvement of heat | Longer time, high energy, expensive |
Electrospinning (Kishan & Cosgriff-Hernandez, 2017) | Good porosity, perfect architecture | Lack of cellular infiltration |
Bioprinting method (Huang, Zhang, Gao, Yonezawa, & Cui, 2017) | Affordable and higher efficiency | Depends on the cells |
Thermal induced phase separation methods (Nam & Park, 1999) | Controllable, Interconnected pores | Can be used only for thermoplastic |
Stereolithography (Melchels, Feijen, & Grijpma, 2010) | High resolution, smooth surface and processing is fast | Toxic resin, Expensive and high temperature |
Fused deposition model (Kamboj, Ressler, & Hussainova, 2021; Krishani, Shin, Suhaimi, & Sambudi, 2023) | No requirement of solvent | High temperature and filament required |
Solvent casting and Particle leaching (Thadavirul, Pavasant, & Supaphol, 2014) | High porosity, lower cost for production | Irregular shaped pores, poor inter connectivity |
Gas foaming (Harris, Kim, & Mooney, 1998) | Low temperature, avoids usage of cytotoxic solvent | Closed pores, long procedure |
Pore Size
By modifying the molecular weight of the employed polymer molecular-weight distribution and architecture in the solution, the properties of the polymer solution like viscosity, conductivity and surface tension (Grenier et al., 2019; Haugh, Murphy, & Brien, 2010) the pore size can be adjusted. Process parameters can also be altered in the electrospinning technique for obtaining the desired pore size are the electric potential, the flow rate of the polymer through the needle, the distance between the needle's tips and the shape of the collector, temperature, humidity and air velocity (Rnjak-Kovacina & Weiss, 2011). Freeze drying method technique is one of the most commonly used method for the preparation of scaffolds. The scientific findings demonstrate that the freezing temperature was lowered, leading to the reduction in the pore size of the scaffold. Additionally, it was discovered that the addition of an annealing step while freeze-drying caused a notable increase (40%) in pore size (Haugh et al., 2010). The range of 100 to 400 µm is the perfect line for scaffold pore size. Under static seeding circumstances, pores greater than 500 µm are too large as to allow the cell and scaffold to come in contact; as a result, cells pass through the scaffolds without adhering to them (Nam, Lee, Khan, & Park, 2020).
Biodegradability
When grafted into living organisms, scaffolds should eventually degrade chemically or enzymatically because they only serve as a non-permanent platform for the growing cells or tissues. Biodegradability is how quickly the scaffolding materials are being degraded (Chocholata, Kulda, & Babuska, 2019). The rate at which the scaffold degrades should ideally match the rate at which new bone grows or tissues regenerate. Scaffold will be eventually replaced by new tissue through a process known as "creeping substitution" once they have effectively been designed and merged with the host bone (Molina, Malollari, Komvopoulos, & K, 2021). The leftovers non-toxic part of the scaffold will either be recycled as metabolites in additional biochemical processes or depart the living system without endangering any other tissue or surrounding organs (Krishani et al., 2023).
Bioactivity and Biocompatibility
The Bioactivity of scaffolds implies the capacity of scaffold to communicate with the created tissue's biological components and the environment (Nitti et al., 2021). It must also be extremely biocompatible for cell growth and adhesion showing minimum immune response in order to avoid severe inflammatory responses that could slow healing and cause the transplanted scaffold to be rejected. There are proven studies in Null mice that wound healing is independent of inflammatory cells and this inflammatory phase can be skipped (Martin et al., 2003). Bioactive scaffolds are created to prevent processes like scarring and to promote the best migration of cells or differentiation, regeneration of tissue or neo-tissue development, and their integration with the host. Traditional passive biomaterials, in comparison, often pose little to no environmental interactions (Roseti et al., 2017).
Mechanical Properties
Materials used for the scaffolds must possess intrinsic mechanical properties resembling those of the local tissues or bones at the anatomical site of implantation (Chan & Leong, 2008) (Chan & Leong, 2008). It offers mechanical support, structural stability, and helps to reduce the risk of stress shielding, implant-related osteopenia, and refracture that follows. The scaffold must also be sufficiently rigid to allow for surgical access for transplantation. Elastic modulus, fracture toughness, tensile strength, fatigue, and elongation percentage are a few examples of the mechanical qualities (Gurumurthy & Janorkar, 2021). The traditional methods for describing a scaffold's mechanical characteristics include compressive and tensile testing. The important obtained values are toughness Young's modulus, and compressive/ tensile strength. The literature states that the skin's range of Young's modulus is 0.42 MPa to 0.85 MPa.
There are various techniques known from the previous literatures that can be used for the fabrication of scaffolds, Table 5 represents some of the techniques used in the fabrication of scaffolds along with their advantages and disadvantages. From the current literature review, it is clearly evident that the most common technique involved in the fabrication of scaffolds are Freeze drying and electrospinning. The structure and characteristics of the resulting collagen scaffold can be accurately controlled by freeze drying and by carefully controlling the growth of the ice crystals. This is accomplished by adjusting a cycle of freezing rather than drying components (Brougham et al., 2017). This hasn't always been fully understood over the years, the focus has been on managing the sample's efficient sublimation rate and understanding the drying phases, where the vacuum is introduced. According to current knowledge, the focus point of any scaffolds, where users attempt to alter the ice crystal structure and porosity of a specific sample should be attained at the first freezing stage.
Freeze Drying
Other names for the freeze-drying procedure include lyophilization and ice templating. The three steps in this method are sublimation, solidification, and dissolution (Ho et al., 2004). First, the solvent is used to dissolve the chosen polymer. The resulting mixture is then poured into a mould and placed in the freezer to harden or freeze. The process can then be finished by mechanical refrigeration, dry ice in aqueous methanol, or liquid nitrogen, allowing it to cool either naturally or chemically. Keeping the temperature under control is important at this stage to prevent the growth of larger crystals that could subsequently damage the scaffold's characteristics (Capuana, Lopresti, Pavia, Brucato, & Carrubba, 2021). Thirdly, the frozen component is subjected to the sublimation process to eliminate water and other solvent molecules. This method is ideal for creating scaffolds with numerous pores, which promote vascularization and aid cell growth and cell differentiation (Fereshteh, 2018). Combining the lyophilization process with salt leaching, gel casting, gas foaming and liquid dispensing methods will improve the properties of the scaffold (Kardan-Halvaei et al., 2023).
Electrospinning Technique
It is a straightforward process where high-voltage electricity is passed through solutions to create fibres (Jun, Han, Edwards, & Jeon, 2018). The interaction between electrostatic repulsion and the surface tension of the charged liquid that experiences significant decreases in voltage is the key idea behind this technology. A Power supply unit, a metallic needle, a syringe pump and a grounded collector are the machine's four main components. This method is frequently used to create nano-fibrous scaffolds (Zulkifli, Nordin, Shaari, & Kamarudin, 2023). The syringe pump's capillary tube receives the liquid injection. The electric field generated by a high-voltage power source exerts muscle strength that raises the liquid's surface tension when it emerges from the metallic needle's nozzle (Zhang, Lv, Lu, Jiang, & Lin, 2015). Additionally, electrostatic repulsion continuously whips the liquid jet, which is then collected as fibers in the grounded collector. Good porosity, aligned fibres and patterned architecture are characteristics of electrospun scaffolds that support cellular response and enhance cell regeneration (Hong, Yeo, Yang, & Kim, 2019). The electrospinning technique has limitations too i.e., it is difficult to precisely manage fiber creation, homogenous cell dispersion, and lack of cellular infiltration (Muthukrishnan, 2022).
Conclusion
From the overall review performed, it is seen that 70% of the research articles published have worked on bone repair using fish collagen-based scaffolds. And there are no direct fish collagen-based products available as a scaffold for direct treatment in patients. Irrespective of so much research work carried out over the past three decades, there is no successful output emphasizing scaffolds with fish-based biomaterials. On the other hand, fish-based products that are already available in the market and other biomaterials like mucus and oil from fish sources are known to have a high wound healing rate. Therefore, there is a need right away to combine scaffolds with fish mucus or oil in scaffolds which would furthermore enhance the wound healing efficiency when merged together. This could be a novel way out in finding a solution for people suffering from diabetes and burn wounds. Hence, there is an urgent need for good quality research to be carried out in this particular area to boost up the market and industries to come out with a quality product in the field of wound healing processes.
Abbreviations
PRISMA: Preferred Reporting Items for Systematic review and Meta-Analysis
MeSH: Medical Subject Healings
ECM: Extracellular Matrix
SYRCLE: Systematic Review Centre for Laboratory Animal Experimentation
WBC: white blood cells
Author contributions
This article was produced through collaboration between the authors. Conceptualization, M.P and B.B.; writing original manuscript, G.M, and B.B.; Selected bibliographic sources, A.M., J.P., A.C.; Review and editing, G.M., A.M., J.P., A.C.; B.B., M.P; All authors have read and agreed to the published version of the manuscript
Conflicts of interest
Given his role as Associate Editor, Balamuralikrishnan Balasubramanian has not been involved and has no access to information regarding the peer review of this article. Full responsibility for the editorial process for this article was delegated to Assocate Editor Si Mi. The authors hereby declare that they have no conflict of interest and have no known competing financial interests or personal relationships that could have appeared to influence the work reported in this paper.