Introduction
Heat stress is among the most important abiotic stresses that affects crop production. According to a temperature study (Figure 1) performed by scientists at the National Aeronautics and Space Administration (NASA) and Goddard Institute for Space Studies (GISS), the earth’s average temperature has increased by about 0.8 °C globally since 1880 (Chen et al., 2014; Guo & Chi, 2014; Raliya & Tarafdar, 2013; Real et al., 2017; Sánchez-Lugo, Morice, Berrisford, & Argüez, 2017; Zhao et al., 2017). While, tremendous rise in warming has been witnessed since 1975, roughly at a rate of 0.15-0.20 °C per decade. In March 2016, the seventh highest global land surface temperature was recorded 1.49 °C above average (NOAA, 2019). This trend of continuous temperature rise is emerging as an alarming issue especially with respect to the global crop production. According to the National Academy of Science, each °C rise in global mean temperature would, on an average, reduce the global yield of wheat, rice, maize and soybean by 6.0%, 3.2%, 7.4%, and 3.1% respectively (Zhao et al., 2017). Based on a mathematical model, the crop production in Southern Africa and Southeast Asia is estimated to be most affected by climate change (Fischer & Edmeades, 2010; Nelson et al., 2009).
Figure 1
Year-to-date global temperatures for 2019 and the ten warmest years on record (https://www.ncdc.noaa.gov/sotc/global/201901)
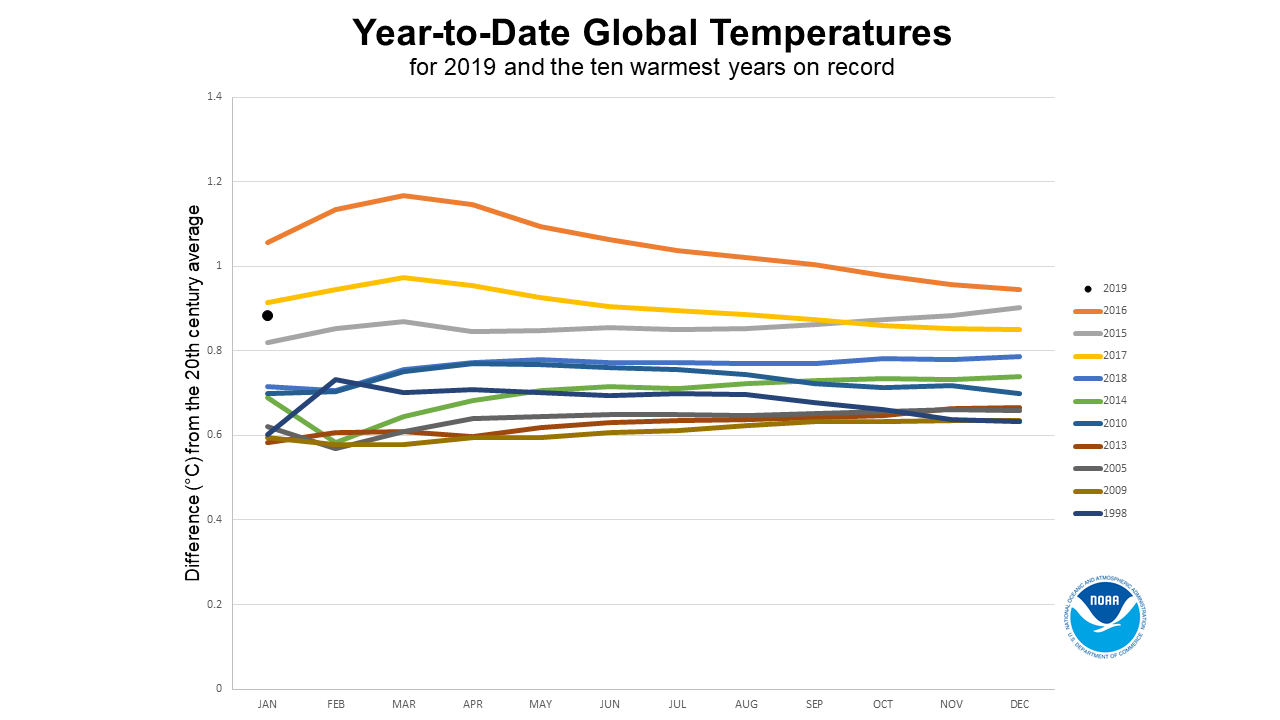
Heat stress leads to irreversible damage to plant growth and development. The damage may range from stunted growth because of severe cellular injury to wilting and plant death caused by the collapse of cellular organization. Usually, the effects of heat stress are more during the reproductive phase as compared to the vegetative phase (Wang, Gan, Clarke, & Mcdonald, 2006). Rise in temperature also impacts pollen fertility as well as plant photosynthetic ability (Zinn, Tunc-Ozdemir, & Harper, 2010). The drop-in photosynthetic activity due to oxidative damage to chloroplasts ultimately reduces the plant dry matter and grain yield (Khodarahmpour & Choukan, 2011; Kim & Lee, 2019). Heat stress also negatively affects the relative water content (RWC), membrane stability index (MSI), chlorophyll a (chl a), chlorophyll b (chl b) and total carotenoid content (TCC). The heat tolerant plants are adept with different mechanisms and processes to fight the heat stress such as regulating vital genes, managing numerous physiological and biochemical adaptations and so forth (Jha, Bohra, & Singh, 2014; Siddiqui et al., 2015). The genotypes equipped with the expression of chaperone proteins are best in enduring the heat stress as they protect other cellular proteins from damage (Modarresi, Mohammadi, Zali, & Mardi, 2010).
Conventional breeding techniques along with the physiological and biotechnological tools could help in selection and development of heat tolerant genotypes (Table 1) with better grain yield (Singh, Handa, & Manchanda, 2021). However, heat tolerance is a multifaceted response achieved from many quantitative and qualitative traits. The genes responsible for these traits are located far apart from each other and amassing each one of the gene in a single genotype is cumbersome and time intensive process. Recently, the use of NPs to improve plant growth and yield after heat stress has been reported (Table 2). The NPs characterization techniques and factors responsible for their uptake and translocation can be better understood by framework figure (Figure 2). The NPs characteristics like their small size, high surface area and stability under high temperature categorically enhance the biochemical and physiological processes in the plants. The small size of NPs permits better penetration into targeted tissues and easier translocation to respective intracellular compartments (Liao et al., 2010). They can effortlessly pass through the cellular membrane and associate themselves with biomolecules and cellular structures. The high surface area of NPs helps in carrying higher concentration of compounds and contributes towards their slow as well as steady release at the site of action (Sindhura, Prasad, Selvam, & Hussain, 2014). Superior bioavailability, catalytic efficiency, dissolution and adsorbing ability make NPs more efficient than their conventional counterparts (Albanese, Tang, & Chan, 2012; Chaudhry & Castle, 2011; Franklin et al., 2007; Ma, Geiser-Lee, Deng, & Kolmakov, 2010; Nair et al., 2010; Navarro et al., 2008; Rico, Majumdar, Duarte-Gardea, Peralta-Videa, & Gardea-Torresdey, 2011). However, the intrinsic properties of the nanomaterial and their functional activities are intensely affected by their particle size, shape, charge, composition, crystalline structure, and morphology.
Table 1
Heat tolerant genotypes in crop plants
Table 2
Impact of nanomaterials on morphological,physiological and biochemical parameters of plants
Characterization of NPs
The intrinsic properties of NPs are largely governed by their nanometer dimensions and materials used for their production. These materials may vary from micelles to metal oxides, from synthetic polymers to biomolecules or combinations thereof. The characterization of NPs is fundamental for assessing their impact on plants and understanding their mode of action. A variety of analytical techniques based on spectrometry and optical principles such as microscopy imaging, chromatography, solid-state Nuclear Magnetic Resonance (NMR), X-ray fluorescence and absorbance among others are favored for NPs characterization (Table 3). The information on the NPs dimensions, size distribution, shape, dispersion, surface charge and porosity generated from these techniques are subsequently useful in understanding plant-NPs interactions. Owing to the complexity of the biological system, NPs are often probed using a number of techniques in combinations to generate complementary information.
Transmission Electron Microscopy (TEM) /Scanning Electron Microscopy (SEM)
Both TEM and SEM use an electron beam to generate images of thin NPs samples. They capture the metal NPs with high electron densities easily as opposed to the amorphous compounds, therefore they are used in a large number of studies involving metal NPs. SEM detects the reflected electrons from the near-surface region while TEM detects the electrons passing through a sample. Both generate a well-defined and precise information about the NPs size, shape, distribution, morphology, and dispersion or aggregation state etc. These properties of NPs contribute towards their in vitro uptake and subsequent localization assessment (Laborda et al., 2016).
SEM and/or TEM have been utilized for the topological study of NPs of magnesium oxide (MgONPs) (Jhansi et al., 2017), gold (AuNPs) (Wali et al., 2017), copper oxide (CuONPs) (Zhao et al., 2017), cerium oxide polymer (nCeO2NPs) (Vinković et al., 2015; Yang et al., 2007; Zhang et al., 2017) and silver (AgNPs) (Arassu, Nambikkairaj, & Ramya, 2018; Li et al., 2017; Nirmala & Pandian, 2015; Prashanth, Menaka, Muthezhilan, & Sharma, 2011; Pyrz & Buttrey, 2008; Roy & Barik, 2010; Thangavelu et al., 2018). The morphological and topological information obtained from SEM/TEM helped in locating NPs in plant and subsequently understanding their mechanism of uptake (Vinković et al., 2015; Yang, Pan, Wang, & Zhao, 2017; Zhang et al., 2017; Zhao et al., 2017), biotransformation (Arassu et al., 2018; Li et al., 2017; Vinković et al., 2015; Yang et al., 2017; Zhang et al., 2017; Zhao et al., 2017), interaction with plant biomolecules (Pyrz et al., 2008; Thangavelu et al., 2018; Zhao et al., 2017) and their further impact on plants. Under current environmental concerns, the SEM/TEM studies are necessary towards the full-scale assessment of NPs risk/benefit ratio.
Table 3
Techniques used for NPs characterization featured in this review
[i] SEM: Scanning Electron Microscopy; TEM; Transmission Electron Microscopy;STEM: Scanning Transmission Electron Microscope; DLS: Dynamic Light Scattering;EDS or EDX: Energy-Dispersive X-ray Spectroscopy; XAS: X-ray Absorption; XRF:X-ray Fluorescence; XRD: X-ray Diffraction; μCT: X-ray ComputedmicroTomography; STXM: Scanning Transmission X-ray Microscopy; Nano SIMS: NanoSecondary Ion Mass Spectrometry; ICP-OES: Inductively Coupled Plasma - OpticalEmission Spectrometry; ICP - MS: Inductively Coupled Plasma - MassSpectrometry; SP-ICP-MS: Single Particle Inductively Coupled Plasma - MassSpectrometry; AFM: Atomic Force Microscopy; TAM: Transient AbsorptionMicroscopy; PHI: Photothermal Heterodyne Imaging; SMS: Spatial ModulationSpectroscopy
Scanning Transmission Electron Microscope (STEM)
STEM combines the principles of SEM and TEM to obtain high resolution NP structure and can be performed on either of the instrument. STEM scans a very fine focused electron beam across the sample surface as well as the transmitted beam of electrons from the sample for nano and atomic scale structural characterization of thin biological cells and materials. In addition, STEM improves spatial resolution of the sample by detecting other signals such as secondary electrons, scattered beam electrons, characteristic X-rays, and electron energy loss, among others. STEM is also suitable for combining annular dark-field (ADF) or High angle annular dark field (HAADF) mapping and spectroscopic imaging to discern the elements of high atomic number (Au, Ag, etc.) from the major elements like C, O, N, etc. in organisms (Hendrickson, Safenkova, Zherdev, Dzantiev, & Popov, 2011; Liu, 2005; Yan & Chen, 2018).
ADF-STEM was used to study the interface arrangement between Au and CeO2NPs while HAADF STEM has been utilized in the identification of silver sulfide nanoparticles (Ag2SNPs) in the sewage sludge. The AuNPs size ranged from < 2nm to 20 nm and high dispersion of only NPs with < 2 nm was observed on the CeO2 because of their easy diffusion onto the CeO2 surface and a long perimeter interface between Au and CeO2 (Akita et al., 2004; Akita, Okumura, Tanaka, Kohyama, & Haruta, 2005). The Ag2SNPs were reported in the 5-20 nm size range with ellipsoidal loosely packed aggregates and the formation of Ag2SNPs may have been caused by the reduced, S-rich environment during the sedimentation process (Kim, Park, Murayama, & Hochella, 2010).
Dynamic Light Scattering (DLS)
DLS technique utilizes the fluctuation in scattering intensity from Brownian movements of dispersed particles to calculate the average NPs size and their aggregation state in a time-dependent manner (Yan et al., 2018). DLS can easily analyze samples having diversity in size (2-3000 nm) and aggregation state as well as the amount (<0.01%) of particles. Due to this DLS could be used to study homogeneity of nucleic acids, proteins, and complexes of protein–protein or protein–nucleic acid, as well as to study protein–small molecule interactions (Brar & Verma, 2011; Stetefeld, Mckenna, & Patel, 2016). DLS study of pepper plants indicated a bimodal volume size distribution of AgNPs in ultrapure water used for watering and established the role of of cis-zeatin in nanostressed plants (Vinković et al., 2017).
Energy-Dispersive X-ray Spectroscopy (EDS or EDX)
The EDS integrated with SEM estimates the unique surface elemental composition and their proportion at different positions of the given sample by measuring X-rays emitted from the surface of the sample (Yan et al., 2018). The EDS imaging is used to determine NPs transportation and biotransformation within the plants. Through EDS analysis, the location as well as biotransformation of CuONPs and CeO2NPs were identified that in turn helped in understanding NPs transportation mechanism (Ma et al., 2017; Zhao et al., 2017).
X-ray Absorption (XAS and X-ray Fluorescence (XRF)
X-ray spectroscopy techniques, XAS and XRF, produce images due to photo-electric effect either from X-ray absorption or emission. XRF is nondestructive and requires no sample preparation whereas XAS requires minor sample preparation. Both techniques are used to determine NPs concentration as well as their respective in situ localization. In addition, XAS could identify very high levels of spatial resolution, information about the oxidation state, electro negativity and NPs coordination using the near edge spectra of XAS (i.e. X-ray absorption near edge structure or XANES) (Zhao et al., 2017). XRF is mostly used for identifying morphology, isotope ratios, quantitative and solid-state speciation. Generally homogenous samples can be analyzed in bulk whereas a heterogeneous and complex sample can only be analyzed after decreasing the beam size that enhances the lateral resolution of NPs. This is subsequently known as μ-XAS and μ-XRF. Both XAS and XRF imaging, characterized NPs morphology and biotransformation in CeO2NPs, CuONPs, CuNPs and AgNPs (Peng et al., 2017; Stegemeier, Colman, Schwab, Wiesner, & Lowry, 2017; Zhang et al., 2017; Zhao et al., 2017).
X-ray Diffraction (XRD)
XRD illustrates information such as the crystal structure, preferred orientation (texture), crystalline phase, average size of crystallite and the strain of crystalline lattice determined through monochromatic beam diffraction at various angles. It is a nondestructive technique used only for crystalline lattice but not for amorphous material. Well-defined wavelength of high intensity is typically incorporated in Synchrotron-based X-ray diffraction (SR-XRD) technique for detecting minor constituents of samples (Lombi & Susini, 2009; Zhao et al., 2017). XRD confirmed the crystal structure of CeO2NPs having arrangement similar to cubic fluorite with an average primary particle size of 28nm (Yang et al., 2017).
X-ray Computed micro-Tomography (μCT)
μCT is also a nondestructive technique and utilizes the principle of tomography at micron level. Digital 3D image is created by passing high flux, monochromatic X-ray beam at various (0° to 180°) angles from the sample. Multiple absorption projections generate highly accurate spatial resolution of NPs in sample (Landis & Keane, 2010; Zhao et al., 2017). 3D visualization of wheat root after AgNPs exposure using μCT and Nano-CT confirmed the presence of AgNPs in the root epidermis and between adjacent root epidermal cells respectively (Real et al., 2017).
Scanning Transmission X-ray Microscopy (STXM)
STXM scans the transmitted X-ray intensity from a small spot to accomplish in situ element mapping at the high lateral resolution of 10-30 nm. The X-ray beam is focused by a zone plate onto this spot. STXM is generally preferred in the studies related to the organic and inorganic phases of mainly carbon/oxygen and metal edges respectively. In addition, it is also utilized in biology, polymer science and catalytic reactions (Groot, Smit, Schooneveld, Aramburo, & Weckhuysen, 2010; Zhao et al., 2017). STXM-XAS, an integrated XAS with STXM microscope, can be used for chemical speciation determination of up to 20 µ thick samples. STXM confirmed the presence and biotransformation of CeO2NPs, Cu-citrate and CuONPs, ytterbium oxide nanoparticles (Yb2O3NPs) and ytterbium chloride nanoparticles (YbCl3NPs) in root cells, intercellular spaces and middle lamella in plants (Peng et al., 2017; Zhang et al., 2012; Zhang et al., 2012).
Nano Secondary Ion Mass Spectrometry (Nano SIMS)
SIMS is considered as one of the most powerful techniques for quantitative investigation of elemental and molecular distributions within the sample. It works by removing particles from the top atomic layer of sample surface by energetic ion beam bombardment causing the discharge of secondary ions that are mapped for elemental distribution determination. NanoSIMS is based on SIMS and has a high mass as well as nanoscopic scale resolution and therefore can be used for detecting most of the periodic table elements (i.e. Hydrogen to Uranium) and their isotopes in minor amount (Zhao et al., 2017; Zhao, Moore, Lombi, & Zhu, 2014). Nano-SIMS confirmed the presence of AgNPs into the periplasmic space and various forms of AgNPs along with silver thiolates in the cell cytoplasm of Chlamydomonas reinhardtii (Wang, Lv, Ma, & Zhang, 2016).
Inductively Coupled Plasma (ICP) based techniques
ICP based techniques use plasma source as electrical discharge to cause atomization or excitation of sample molecules. Subsequent to atomization, spectrometers determine the NPs concentration, number and elemental composition among others. ICP based techniques can quantitatively analyze solid, liquid or suspension samples (Laborda et al., 2016; Zhao et al., 2017).
Inductively Coupled Plasma - Optical Emission Spectrometry (ICP-OES)
ICP-OES is considered as one of the optimal methods for nutrient detection in soil and contaminants in food. The atomization of free atoms emits electromagnetic radiation of particular wavelength by specific element and subsequently measured by OES to determine elemental concentration. This technique is also known as Inductively Coupled Plasma Atomic Emission Spectrophotometry (ICP-AES) (Zhao et al., 2017). CuNPs in the aleurone layer of unpolished grain and high Cu content in roots as compared to shoot was reported (Peng et al., 2017).
Inductively Coupled Plasma - Mass Spectrometry (ICP - MS)
In ICP-MS, the samples are first decomposed, atomized and ionized. The ions are subsequently detected and sorted by MS according to their mass-to-charge ratio. This technique is used for tracing, categorization, and quantification of metal and non-metal NPs and their isotopes (Zhao et al., 2017). ICP-MS has simpler, multi-elemental, low detection and high precision spectra and therefore frequently used in biological, geochemical and environmental studies. The nCeO2 purity and concentration as well as their translocation mechanism was confirmed by ICP-MS (Yang et al., 2017).
Single Particle Inductively Coupled Plasma - Mass Spectrometry (SP-ICP-MS)
SP-ICP-MS identifies the minuscule levels of elements by measuring an individual ionized particle, one at a time, during a short window of 100µs known as dwell time. Swift and continuous data acquisition in dwell time explores precise size determination as well as NPs accumulation, localization, transformation and possible uptake kinetics in plant tissues (Zhao et al., 2017). AuNPs translocation and AgNPs uptake along with biotransformation in tomato, soyabean and rice were reported using SP-ICP-MS (Dan et al., 2015; Li et al., 2017).
Atomic Force Microscopy (AFM)
AFM collects three-dimensional topographical information about NPs by focusing a flexible cantilever beam through physical contact between the sample and the mechanical probe using feedback system. The deflection of the cantilever beam is in proportion to the force of interaction between the sample and the probe (Rao et al., 2007). Additionally, chemical potential, electric and magnetic properties, among others are also measured after modifying probe tip. The topological information of AgNPs and AuNPs synthesized using plant extract were observed by AFM (Gopinath, Gowri, Karthika, & Arumugam, 2014; Pasca et al., 2014; Rashmi & Sanjay, 2016).
Transient Absorption Microscopy (TAM)
TAM is a label-free imaging technique used for non-fluorescent semiconducting and metallic molecules with a potential in biomedical applications. Two collinear wavelengths, primary of 1032 nm known as pump and secondary of 810 nm known as probe, are utilized for the atom excitation. TAM utilizes the time delay between them for further imaging and analysis (Tong et al., 2012). Gold nanorods (AuNRs) were observed in living cells without any hindrance from auto fluorescent background and therefore can be used as probes in various biological applications (Chen et al., 2014).
Photothermal Heterodyne Imaging (PHI)
PHI is a highly sensitive and simple technique used for detecting the absorption of frequencies in the scattered field generated through single beam probe after interacting with time-modulated variation of the refraction index around non fluorescent nano-objects (Berciaud, Cognet, Blab, & Lounis, 2004). It offers unparalleled advantages for studying, identifying or detecting NPs as metallic clusters containing only 67 atoms and it can be employed to track NPs in cellular biomolecules (Berciaud et al., 2005). The blinking, photo bleaching, fluorescing and scattering backgrounds doesn’t affect the PHI imaging and mapping. The cytosolic dynamics of individual AuNPs in non-luminescent semiconductor nanocrystals were analyzed by PHI (Berciaud, Lasne, Blab, Cognet, & Lounis, 2006).
Spatial Modulation Spectroscopy (SMS)
SMS utilizes the strict in and out movement of single NP from the area of laser beam focus to gain information of NPs having smaller size than laser focus. The focus position is repeatedly modulated and updated with regard to NP position. At the same time a photo detector and a lock-in amplifier detect the transmitted light. This method is employed for determining polydispersity with a broad range of sizes in carbon nanoparticles (CNPs) and AuNPs (Devkota, Devadas, Brown, Talghader, & Hartland, 2016; Kollmann et al., 2018). SMS setup found AuNP bipyramids for optical response quantification in a liquid environment (Rye, 2017). This offers high potential in label free biosensing and biomedical related assays via metallic and nonmetallic NP imaging.
NPs Uptake and Translocation in Plants
There is a lack of constant and generally applied research related to the effects of specific NP with respect to their transport. This lack further leads to the major hole not only in understanding the uptake mechanism, but also in their subsequent distribution (Chouhan & Mandal, 2020; Nevius, Chen, Ferry, & Decho, 2012; Tombuloglu et al., 2020). The NPs chemical, physical and dosage properties among others play a major role in their transport through cell wall and cell membrane. Surface chemical reactions like ion exchange, surface precipitation and physical adsorption are identified as some of the most common processes for NPs uptake. The significance of NPs size, magnitude, and zeta potential are also established in determining their transport inside the plant (Gupta & Rastogi, 2008; Srividya & Mohanty, 2009). The first plant-NPs interaction takes place at the plant cell wall. Numerous pores having diameter from 5-20 nm present on the plant cell wall are responsible for the plant sieving properties and regulate the particle movement. The NPs having diameter within the range easily enter into the plant cell, whereas the NPs of larger diameter interact with the cell wall proteins and polysaccharides, consequently forming new pores and routes for their entrance. The Zinc oxide nanoparticles (ZnONPs) reportedly enhanced the permeability of bacterial cells by the formation of new pores (Brayner et al., 2006; Nair et al., 2010; Navarro et al., 2008).
Surface sorption processes like ion exchange, chelation, chemical precipitation and endocytosis (Aslani et al., 2014; Kurepa et al., 2010; Maine, Duarte, & Suñé, 2001; Tani & Barrington, 2005) as well as ion transporters such as carrier proteins, aquaporins, and ion channels (Somasundaran, Fang, Ponnurangam, & Li, 2010; Yadav, Kumar, Kumari, & Yadav, 2014) have been observed as an alternative for metal NPs uptake by plants. A mathematical model called lipid exchange envelope penetration (LEEP) based on the theory of lipid exchange between the NPs and the cell envelope aids to our understanding of the NPs penetration into the cells (Wong et al., 2016). Even though there is a dearth of comprehensive research in NPs, still a probable overall mechanism can be drawn as shown in Figure 3. NPs uptake and distribution by plants first involves their uptake through plant leaves or roots and their subsequent translocation via xylem and the phloem system.
Foliar Uptake
Substantial advancement to understand the mechanism and pathways involved in foliar uptake has been established from both basic and applied research such as foliar leaching of nutrients, foliar fertilization, and foliar uptake of non-gaseous pollutants among others. Two most prominent foliar uptake pathways are cuticular and stomatal. The cuticular pathway involves two parallel routes of uptake, i.e. lipophilic and hydrophilic. The lipophilic pathway entails diffusion in cutin and waxes by lipid loving apolar and noncharged molecules, whereas, the hydrophilic pathway involves dissolution of water loving polar or ionic molecules through aqueous pores of 0.6 to 4.8 nm diameter. The dissolution typically depends on the humidity as well as the solubility and hygroscopicity of molecules. The aqueous pores are generally found over both cuticular ledges and the anticlinal walls of stomatal guard cells. The stomatal pathway is a solid-state pathway enabling the uptake of the suspended and hydrophilic molecules via diffusion. Compared to outer cuticular layer, the cuticle over the sub-stomatal chamber was found to be thin and might be a key to incidences for stomatal pathway This pathway has a high transport velocity and is based on the size exclusion limit of molecules over 10 nm in diameter (Eichert, Kurtz, Steiner, & Goldbach, 2008; Fernández & Eichert, 2009; Uzu, Sobanska, Sarret, Munoz, & Dumat, 2010).
The AgNPs entrapment by cuticle and their oxidation in addition to complex formation with thiol molecules preceding to their entry into the leaf tissue through stomata was reported (Eichert et al., 2008). Micrometer-scale AgNPs agglomerates were observed on the leaves surface as well as within the leaf tissues including epidermis, mesophyll and vascular tissues. This could be inferred as the deposited AgNPs on the leaves surface first crossed the epidermis and eventually transferred to the leaf tissues. A similar accumulation of AuNPs, TiO2NPs, CeO2NPs and PbNPs aggregates after foliar exposure was reported in rapeseed, maize and lettuce. Further, embedded NPs were also recognized in leaf cuticular waxes as well as in the sub-stomatal chambers (Birbaum et al., 2010; Larue et al., 2012). During another study, TiO2NPs were traced in the leaf parenchyma of Arabidopsis after foliar exposure. Likewise, the AgNPs accumulation was observed in stomatal guard cells of Arabidopsis seedlings cotyledons (Geisler-Lee et al., 2014; Larue et al., 2014). The movement of NPs in plants after foliar exposure can be summarized as their first crossing of cuticle or stomata and then reaching epidermis or guard cells. From epidermis or guard cells, NPs make their way into mesophyll or other inner leaf tissues. At last NPs reach the parenchyma or plant vascular system and might possibly be distributed to other plant parts.
Root Uptake
Extensive studies on the plant nutrient as well as water uptake suggested the significance of cellular penetration in these processes, consequently it is also accepted that the NPs uptake through roots might occur in the same manner. The simple diffusion, facilitated diffusion, and active transport are the mechanisms of the nutrient and ion uptake into the root cytosol by establishing an osmotic potential across the membrane. Currently, two well-known pathways for root uptake are symplast and apoplast transport. In symplast transport ions or water utilizes plasmodesmata for moving between cytosol of plant cells. While in apoplast transport, the region of continuous cell wall among cells i.e. apoplast is employed. The apoplast transport is freely permeable to water/ions, but due to the presence of casparian strip water/ions cannot reach across the endodermis via this pathway (Doran, 2013). Considerable progress to comprehend the mechanism and pathways of NPs root uptake has been achieved from several studies on plant-NPs interactions. For the most part, it is revealed that the NPs first adhere to plant roots and their subsequent uptake might be due to the chemical or physical or a combination of various processes. Yet the exact path followed in the root uptake of NPs is not well-known (Aslani et al., 2014).
The AgNPs of 40 nm were found accumulated in and around Arabidopsis root tip regions like border cells, columella cells, root cap, axial/lateral root cap and epidermis. The subsequent study suggested the initial attachment of AgNPs to the Arabidopsis primary roots surface during the earlier stages of NPs exposure and later their entry into the root tips. Afterwards, the gradual movement of AgNPs into root tissues such as lateral root primordia and root hairs was also observed (Geisler-Lee et al., 2012; Geisler-Lee et al., 2014; Schwab et al., 2016; Zhai, Walters, Peate, Alvarez, & Schnoor, 2014). Other studies found that AgNPs in Arabidopsis and AuNPs in the woody poplar plant amassed at plasmodesmata and in the cell wall of the phloem complex in root cells. The possible movement of NPs with diameter <15–40 nm across the plasmodesmata was advocated (Schwab et al., 2016; Zhai et al., 2014). Similarly, the high amount of ZnONPs aggregates was observed previous to their entry into the epidermis and later penetration into the root cortex of corn plants. The presence of a small amount of ZnONPs aggregate was also noted in the root endodermis and later closer to the Casparian strip (Schwab et al., 2016; Zhao et al., 2012).
Decades of research has also pointed towards the role of soil microorganisms and their interaction with the plant in micronutrient availability and accessibility to plants. Most of the symbiotic and pathogenic relationships occur by cell wall elongation; mainly by interaction with mucilage and exudates released by plant into the rhizosphere. The root bacterium Pseudomonas chlororaphis O6 considerably affected the bioavailability of metal nutrients in mung bean after CuONPs and ZnONPs exposure. The plant legumes soybean association with nodule forming nitrogen fixing bacteria Rhizobium leguminosarum was found to be the cause of 22% reduction in Cd accumulation. In the same way, a 12% increase in ryegrass and 14% increase in barley uptake of Cd was observed due to the bacterial or comparable association in non-legumes. The information on the NPs uptake with respect to rhizo-biosphere is still unknown and open to exploration (Belimov & Dietz, 2000; Dimkpa, Mclean, Britt, & Anderson, 2015; Guo et al., 2014; Schwab et al., 2016). Thus, possible routes for NPs uptake through root can be summarized as from the root surface to root border cells subsequently to root cap/root tip to epidermis/endodermis. The NPs can then move through either plasmodesmata or apoplast. The NPs moving through plasmodesmata can further cross cambium and the vascular system of plant whereas the movement of NPs along the apoplast is blocked by the casparian strip from reaching the plant vascular system. The NPs following the apoplast can either cross the plasmodesmata or cell membrane or will be accumulated along the casparian strip. Another way the NPs uptake can be influenced is due to plant interaction with soil microorganism.
Translocation within plants
The essentials of translocation through the plant vascular system are documented, yet the exact path of NPs distribution is not well-known. Two distinct pathways for translocation and distribution in plants are xylem and phloem. The xylem is mainly involved in unidirectional i.e. root to shoot distribution of water, ions and nutrients following the transpiration stream. While, the phloem distributes the product of photosynthesis, amino acids and macromolecules multi-directionally to various heterotrophic organs such as roots, flowers, fruits, and developing seeds. Jointly, xylem and phloem are responsible for supplying the water, nutrients and assimilates, in suitable forms and magnitude, to facilitate growth and development of plant in a controlled and synchronized manner (Atkins & Smith, 2007; Banerjee et al., 2006; Buhtz, Kolasa, Arlt, Walz, & Kehr, 2004; Lough & Lucas, 2006). In similar fashion, NPs transported mainly through the xylem are expected to travel predominantly from root to shoot or leaves. On the contrary, NPs transported mainly through the phloem will probably build up in plant sink organs like fruits, flowers, grains, or young leaves (Lin et al., 2009; Servin et al., 2013). Root-to-fruit translocation of TiO2 NPs in cucumber and root-to-leaves along with root to stem translocation of the carbon nanoparticles (CNPs) in rice was observed. Likewise, potential translocation of the magnetite (Fe3O4) NPs from root to aerial tissues of pumpkin as well as NPs translocation and distribution from root to shoot to root in Arabidopsis was also confirmed (Wang et al., 2011; Yang et al., 2017). During the carbon NPs uptake and translocation in rice, the easy uptake of fullerene C70 by roots and later their transportation to shoots was observed. Additionally, the potential of fullerene C70 translocation by phloem after foliar uptake was also demonstrated. A different study delivered aerosolized AuNPs to watermelon leaves for understanding their uptake and translocation mechanism and detected AuNPs in leaves, stems and roots (Lin et al., 2009; Raliya et al., 2016). On the other hand, a number of studies also reported the absence of NPs translocation after the NPs uptake by plants. For instance, no root to shoot translocation of ceria NPs in maize and ZnONPs in ryegrass was reported after NPs exposure. In similar fashion, absence of nano-anatase Ti2O NPs translocation in rice was reported, even though the uptake of NPs was registered (Kurepa et al., 2010; Lin & Xing, 2007).
Factors affecting NPs Uptake, Translocation and Distribution
Although a full picture of probable NPs uptake, translocation and distribution mechanism can be drawn but a glaring question of which NP will follow which of the uptake and translocation pathway remains unanswered. Both NPs properties as well as the host plant tissues are perhaps responsible for change in NPs uptake and accumulation pattern (Liu et al., 2020).
Size and distribution
The assessment and visualization of NPs as a possible smart delivery system have reported the significance of NPs size in uptake by plant tissue. The probable cause of size-based selection criterion is the presence of cell walls and waxes. On an average, 40 to 50 nm sizes are considered as the exclusion limit for NPs uptake by various plant tissues (Eichert et al., 2008; González-Melendi et al., 2008; Sabo-Attwood et al., 2011; Taylor et al., 2014). The AuNPs of 3.5 nm were detected inside both root and leaf tissues whereas of 18 nm were observed only on the outer root surface in Nicotiana xanthi seedlings. A different study indicated the absence of direct uptake of 5 to 100 nm AuNPs in alfalfa but observed Au oxidation and their subsequent uptake as Au ions. The ZnO granules of lesser diameter were reportedly taken up faster than their larger counterparts though both were similar in weights. The better Zn distribution in smaller granules played a role in higher Zn uptake (Lin & Xing, 2008).
Chemical composition
Numerous studies have indicated the vital role of the chemical composition in NPs uptake and distribution. Out of three different AgNPs species (Ag0, Ag2S and AgNO3), only Ag0 and AgNO3 were reportedly taken up by plant tissue, whereas Ag2SNPs were mapped only to the root surface of duckweed. Later on, the biotransformation of Ag0NPs after moving to plant tissues into silver sulfide and silver thiol species was also established (Stegemeier et al., 2017).
Morphology
The morphology is also responsible for the different outcomes related to NPs uptake and distribution. The watermelon leaves were exposed to AuNPs of different morphology (sphere, cube, rod and rhombic dodecahedra) in the form of droplet and aerosolized NPs suspension. High accumulation of rod shaped and low accumulation of the cube shaped AuNPs was observed through droplet method. On the contrary, the opposite was observed for aerosolized NPs suspension. Overall higher translocation was recorded from aerosolized NPs suspension due to their low aspect ratio as compared to droplet method (Raliya et al., 2016). Surface coating of NPs through tannin and citrate among others also alters their plant absorption and accumulation properties (Judy, Unrine, Rao, Wirick, & Bertsch, 2012).
Surface charge
Another contributing factor responsible for NPs uptake across cell wall and other plant tissues is the surface charge. Few studies have demonstrated a correlation between root uptake/shoot translocation and the surface charge. AuNPs exposure to plant seedlings of rice, radish, pumpkin, and perennial ryegrass showed rapid uptake of positively charged NPs as compared to negatively charged by plant roots. However, higher efficiency of translocation of negatively charged AuNPs was observed from roots to stems and leaves. Similar results have been reported by another independent study in rice (Koelmel, Leland, Wang, Amarasiriwardena, & Xing, 2013; Zhu et al., 2012). Higher uptake and accumulation of positively charged NPs in the plant tissues may be apparently due to the presence of negative charge in the plant cell walls.
Exudates
Mucilage or exudates released by seed cell envelope or plant roots or phytoplankton’s cell surface are also significant for NPs uptake (Driouich, Follet-Gueye, Vicré-Gibouin, & Hawes, 2013; Mcnear, 2013; Schwab et al., 2016; Yang, Baskin, Baskin, & Huang, 2012). Dynamic interactions of NPs with the outer layer of mucilaginous cell surface and phytoplankton debris primarily depends on their size, surface coating and solubility. The TiO2NPs impacted the cell surface morphology and mechanical properties of Anabaena variabilis (Cherchi, Chernenko, Diem, & Gu, 2011). It was suggested that the secreted biomolecules by algal cells acted as a biodispersant to further reduce the size distribution of NPs aggregates. The organic acids released from plant root cells induce the NPs dissolution ensuing in higher NPs uptake as demonstrated by the enhanced dissolution of lanthanum oxide nanoparticles (La2O3NPs) due to acetic acid released by plants. One possible mechanism involves NPs trapping in mucilage for their uptake by plant in a charge independent manner while other involves change in mucilage or exudates discharge due to interaction between plant root cap and NPs surface charge (Driouich et al., 2013; Ma et al., 2011; Rai et al., 2018; Zhang et al., 2011; Zhang et al., 2012). The positively charged NPs has been reported to favor higher exudates production. Extensive NPs amassing and phytoplankton agglomeration due to excess of polyphenolic production was seen in mucilage of the Fucus serratus. The TiO2NPs exposure for longer durations resulted in increased thickness in the mucilage layer in Anabaena variabilis. Similarly, the secretion of extracellular matrix was observed by Isochrysis galbana which forms complex with zero-valent nanoiron (nZVI). The interaction of NPs with mucilage or exudates corresponds with the high NPs accumulation on the root surface and their reduced translocation. Similar properties were shown by positively charged AuNPs, CuONP, AgNPs and TiO2NPs in different plant species like rice, ryegrass, radish, pumpkin, wheat and Chlamydomonas reinhardtii (Cherchi et al., 2011; Kadar, Rooks, Lakey, & White, 2012; Mcmanus et al., 2018; Schwab et al., 2016; Zhang et al., 2011; Zhu et al., 2012). On the contrary, the non-adsorption of negatively charged exudates might be the cause of their higher translocation within plants (Schwab et al., 2016).
Growth medium
Multiple studies have reported the significance of not only the plant species specificity for NPs uptake and further translocation but also of the growth medium/soil used. The pumpkin seedlings grown in three medium viz hydroponic culture, soil and sand presented three different outcomes in Fe3O4NPs uptake. Maximum NPs uptake in the roots, stems and leaves from hydroponic culture whilst no uptake in soil and minimal uptake in sand grown seedlings was recorded. This suggested that the variation in the NPs uptake may be due to the interaction of the NPs to the soil and sand grains (Singh, Singh, Hussain, Singh, & Singh, 2015; Zhu, Han, Xiao, & Jin, 2008). The effect of environmental abiotic and biotic components on NPs assimilation and uptake by plants has been reported. The presence of humic acid and other organic matters in soil led to enhanced NPs bioavailability, whereas, the opposite effect was observed in the presence of salt ions due to the NPs precipitation. The impact of biotic components such as bacteria and fungi may be primarily due to symbiotic relationships (Feng et al., 2013; Navarro et al., 2008; Wang et al., 2016).
Plant species
The physiological differences in plant species is also reflected through the variation in their NPs uptake. The rapid accumulation of the magnetite NPs was detected in pumpkin plants but not in the lima bean. The disparity in uptake was observed for carbon-coated iron nanoparticles (FeNPs) in pea, tomato, sunflower and wheat. Increased buildups of carbon-coated FeNPs in pea plant roots as compared to sunflower and wheat were detected. At the same time, rapid NPs translocation to aerial plant parts was observed in wheat and pea than sunflower and tomato. A different study, recorded the higher AuNPs accumulation in radish and ryegrass roots as compared to rice and pumpkin. In addition, higher uptake of CeNPs was shown in alfalfa and tomato than cucumber and corn (Cifuentes et al., 2010; López-Moreno, Rosa, Hernández-Viezcas, Peralta-Videa, & Gardea-Torresdey, 2010; Zhu et al., 2012; Zhu et al., 2008).
To sum-up, a single factor cannot be pointed out as a major force behind the NPs uptake, distribution and translocation. A combination of NPs size, morphology, concentration, surface charge, surface coating, solubility, and aggregates, among others although concerned/target plant species are crucial for NPs-plant interactions. The method of NPs delivery as well as biotic and abiotic components of rhizosphere are other key players. Therefore, to develop further understanding of the exact NPs mechanisms for their selective uptake by different plant species, all of the factors and combinations needs to be explored comprehensively.
Role of NPs in Heat Stress Tolerance
Heat stress influences plant growth and crop yield by impacting the photosynthesis, one of the most thermosensitive plant functions (Wang et al., 2008). This severely alters many physiological processes such as respiration, membrane permeability and reactive oxygen species (ROS) production (Awasthi, Bhandari, & Nayyar, 2015; Parankusam, Bhatnagar-Mathur, & Sharma, 2017; Prasad, Ferretti, Sedlářová, & Pospíšil, 2016). This has been depicted in Figure 4. Because of the complex nature of the heat tolerance mechanism, scientists are now interested in developing new tools to cope up with the stress without modifying the genetic makeup of the plant. Consequently, studies of trace elements, phytohormones, osmoprotectants, and signal molecules having the potential to protect from heat stress are gaining momentum (Bhatia, Gulati, & Sethi, 2021; Hossain & Fujita, 2013; Mostofa & Fujita, 2013; Nahar, Hasanuzzaman, Alam, & Fujita, 2015; Savvides, Ali, Tester, & Fotopoulos, 2016; Wang et al., 2014). These molecules with their growth promoting and antioxidant capabilities have proven beneficial for plants to combat heat stress (Chan & Shi, 2015; Khan, Iqbal, Masood, Per, & Khan, 2013; Mostofa, Yoshida, & Fujita, 2014; Sagor, Berberich, Takahashi, Niitsu, & Kusano, 2013; Zhao et al., 2020). Enhanced efficiency and bioavailability of these molecules is ensued through nano-emulsions or NPs formation as well as their delivery to target sites (Dhiman, Yadav, Debnath, & Das, 2021; Heffer & Prud’homme, 2012).
Metal or Metal Oxide Based NPs
Silver NPs (AgNPs)
AgNPs are among the most used NPs due to their chemical stability, catalytic activity, and localized surface plasma resonance. Dissolution of silver compounds lead to Ag+ ions dissociation and later gain of an electron from oxidation–reduction reaction resulting in AgNPs formation. The low concentration of (50-75mg/l) AgNPs protected wheat plant against heat stress by improving morphological growth such as plant root length, shoot length, root number, plant fresh and dry weight (Iqbal et al., 2019). These treated plants showed a significant increase in RWC, MSI, chl a, chl b, TCCs, SOD, POX and CAT on experiencing stress. Substantial amplification was also registered in total phenolic content (TPC), total flavonoid content (TFC), ascorbate peroxidase (APX) and glutathione peroxidase (GPX) (Iqbal et al., 2018). AgNPs treatment enhances thermo-tolerance, but the mechanism of AgNPs action needs further investigation at both genome, proteome and metabolome level.
Silicon dioxide NPs (SiO2NPs)
Silicon (Si) is the second most abundant element in soil and has proven beneficial for the healthy growth and development of many plant species. It safeguards plant from biotic and abiotic stresses by decreasing ROS concentration. Si application under high temperature (35°C) accounted for increased activity of antioxidant enzymes such as SOD, APX and GPX though reduced CAT activity was observed (Soundararajan, Sivanesan, Jo, & Jeong, 2013; Xie, Li, Tao, Zhang, & Zhang, 2012). In recent years, the role of foliar application of nano-silicon dioxide (SiO2NPs) has been reported in heat stress tolerance. This improved plant growth and their performance by enhancing the proline accumulation, antioxidant enzyme activity, gas exchange and photosynthetic apparatus efficiency (Kalteh, Alipour, Ashraf, Aliabadi, & Nosratabadi, 2014; Karimi & Mohsenzadeh, 2016; Kim, Khan, Waqas, & Lee, 2017). However, the interaction of Si and plant antioxidant enzyme system remains poorly understood, and further in-depth analyzes are needed. Transcriptomics might play a significant role in understanding the mechanism responsible for the Si-mediated regulation of stress responses.
Titanium dioxide NPs (TiO2NPs)
TiO2NPs promote photosynthesis encountering mild heat stress (Qi, Liu, & Li, 2013). The increased net photosynthetic rate, H2O conductance and transpiration rate were observed after application of TiO2NPs in tomato. A significant reduction in the minimum chlorophyll fluorescence, relative electron transport and non-regulated photosystem II (PS II) besides increased regulated PS II energy dissipation was also reported. Improved biomass production through amplification of metabolic, photo-catalytic and light energy conversion activities turned these NPs into an efficient nutrient source for plants (Gao, Zhou, Zhang, Yang, & Lin, 2008). (Raliya, Biswas, & Tarafdar, 2015) detected a significant increase in shoot length, root length, and root nodule content along with total soluble protein and chlorophyll content in the leaves of moong bean seedlings after TiO2NP treatment.
Selenium NPs (SeNPs)
Selenium (Se) is a trace element that serves as a stress protectant against different environmental stresses including high temperature. The SeNPs application either as a soil supplement or as foliar spray enhanced antioxidant capacity, growth, and yield of the higher plants (Ardebili, Ardebili, Jalili, & Safiallah, 2015; Djanaguiraman, Belliraj, Bossmann, & Prasad, 2018; Hasanuzzaman, Hossain, & Fujita, 2010; Jiang, Zu, Shen, Shao, & Li, 2015; Lyons et al., 2009; Turakainen, Hartikainen, & Seppanen, 2004). Djanaguiraman and coworkers observed that the foliar spray of 75 mg L−1 Se significantly increased the stomatal conductance, photosynthetic, and transpiration rate of sorghum. Moreover, reduced O2−, H2O2, malondialdehyde (MDA) as well as membrane injury were also registered. Even though elemental Se is water insoluble and biologically inert, SeNPs possess prominent bioactivity and listed as safe for plants. The biological and antioxidant properties of SeNPs increase with their subsequent decrease in particle size. The foliar spray of 10 mgL¯1 SeNPs in sorghum decreased the concentration of signature oxidants whereas stimulated the antioxidant enzyme activity. They also facilitated unsaturated phospholipids at higher levels and improved the pollen germination leading to a significant rise in seed yield (Djanaguiraman et al., 2017; Djanaguiraman, Prasad, Murugan, Perumal, & Reddy, 2014).
Cerium oxide NPs (CeO2NPs)
Cerium is a rare earth metal with a wide range of catalytic applications. It has been widely used as a fertilizer additive for improving plant growth. The role of CeO2NPs (nanoceria) has been identified in scavenging ROS and photosynthesis under excess light/dark and heat or cold conditions (Heckert, Karakoti, Seal, & Self, 2008; Korsvik, Patil, Seal, & Self, 2007). Nanoceria with 35.0 % or lower Ce3+/Ce4+ ratio reduces ROS level in leaves and increases the quantum yield of photosystem II, carbon assimilation and Rubisco carboxylation rates. Biological activity of CeNPs was found to be dependent on the presence of Ce3+/Ce4+ redox couple on the NP surface. Currently established CeNP mechanism resembles with metallozymes containing metal ions, such as Fe3+, Cu2+, or Mn3+, in scavenging ROS from cells and tissues. Ce3+ ions reduce superoxide into hydrogen peroxide similar to SOD and are oxidized to Ce4+ (Dowding, Seal, & Self, 2013; Xue, Luan, Yang, Yao, & Zhou, 2011) . This oxidation afterwards eliminates deleterious free radicals, such as hydroxyl (Dowding et al. 2013), and peroxynitrite (ONOO−) (Pirmohamed et al., 2010). On the other hand, the reduction of Ce4+ ions to Ce3+ ions induces hydrogen peroxide oxidation to molecular oxygen, in a manner similar to the catalase enzyme (Djanaguiraman, Nair, Giraldo, & Prasad, 2018).
Nano encapsulated Plant Growth Regulators and Signal Molecules
Plants have different advanced mechanisms to ensure endurance in extreme temperatures. These mechanisms include alteration in physiological parameters like an increased antioxidant activity, the accumulation of metabolites such as heat-shock proteins (HSPs), osmoprotectants, hormones, signal molecules, etc. (Bokszczanin et al., 2013). Since 2007, exogenous use of osmoprotectants, phytohormones and signaling molecules have shown a positive effect on plants under heat stress (Kotak et al., 2007). For instance, Nitric oxide (NO), one of the signaling molecules, interacts with plant hormones to elicit expression of genes effective in overcoming oxidative stress. It demonstrates tolerance to biotic and abiotic stresses as well as a role in various physiological processes like plant growth, stomatal movement, iron homeostasis etc. (Anelia, 2017; Hasanuzzaman, Nahar, & Fujita, 2013; Waraich, Ahmad, Halim, & Aziz, 2012; Zandalinas, Balfagón, Arbona, & Gómez-Cadenas, 2017). Although exogenously applied NO alleviated high temperature induced damages (Hasanuzzaman, Hossain, & Fujita, 2011) still NO released at higher concentration registered toxicity in plants. This was due to lack of thermal and photochemical stability in NO donors. The controlled release of NO from nanomaterials achieved through NO donors’ encapsulation could improve efficiency over their direct exogenous application (Seabra, Rai, & Durán, 2014; Song, Ding, Hao, Sun, & Zhang, 2006). Synthetic (e.g., polycaprolactone, polyacrylamide, polyacrylate (Turos et al., 2007) or natural polymers, (e.g., albumin) (Martínez, Iglesias, Lozano, Teijón, & Blanco, 2011), DNA and chitosan (Bilensoy et al., 2009), poly L-lactide (PLA), and gelatin (Mainardes, Khalil, & Gremião, 2010; Saraogi, Gupta, Gupta, Jain, & Agrawal, 2010) are generally utilized for nanomaterial encapsulation. Site directed application of NPs achieved through encapsulation not only reduces expenses but also prevents leaching of unused chemicals into the environment (Grover, Singh, & Venkateswarlu, 2012).
Plant hormones such as abscisic acid (ABA), salicylic acid (SA), jasmonic acid, gibberellins, auxins and cytokinins also play a significant role in imparting heat tolerance. Heat tolerant plants synthesize ABA at an elevated level under heat stress relative to heat sensitive plants (Ding, Song, Wang, & Bi, 2010). Apart from ABA, involvement of SA in heat-stress responses elicited by plants has also been observed. SA reportedly stabilizes the trimers of heat shock transcription factors and facilitates their binding to respective promoter sites. Similarly, epibrassinolide treatment during the heat stress also directly impacts the protein synthesis. Both SA and epibrassinolide enable plants to withstand heat stress by the rapid and elevated synthesis of heat shock proteins (Kagale, Divi, Krochko, Keller, & Krishna, 2007). Chen and coworkers studied the effect of 50μM JA solution on grape seedlings and observed tolerance under heat stress (42°C). However, due to short half-life these plant hormones can only impart limited tolerance. Plant hormones can also be encapsulated, in the same manner as NO, for achieving better tolerance in heat sensitive plants (Chen, Yu, Zhan, & Kang, 2006).
Future prospective and Conclusion
Nanotechnology in agriculture could be fundamental in various global predicaments, from addressing the impact of climate change to enhance bioavailability of plant nutrients and many more. Both target specific and non-target specific approaches of nanotechnology are significant for agriculture. The use of target-specific NPs can reduce the damage to non-target plant tissues and the amount of chemicals released into the environment. However, various aspects of nanotechnology are still untouched such as Nano-agropesticides, nanofertilizers, and nanoherbicides. and Nano-nutraceuticals. The interactions between plants and NPs provide real hope for attaining agriculture sustainability, specifically in relation to onsite detection of pathogens, and crop improvement.
In any case, thorough research is needed to comprehend physiological, biochemical, and molecular mechanisms of NPs on plants. Additionally, the synthesis of nanomaterials itself currently is a noteworthy issue as controlling the number of active atoms on the NPs surface accurately is challenging. New strategies for the NPs production ensuing definite composition, uniform surface modification, reproducible action, etc. are necessary. NPs action reproducibility is directly co-related to their purity, stability and disposability. The study of substrates’ physical and chemical properties is another need of the hour and might help in creating successful NPs. However, even before creating successful NPs, the understanding of their entry points/routes in the plant system as well as the mode of action is required. Recent NPs studies not only focus on different pathways and biomolecules interactions, but also their effects on different genes along with issues of risk associated with NPs usage in plant system. Furthermore, the studies are needed to scale up the production processes and lowering costs of NPs. All in all, nanotechnology in a vast field and much more needed to be done in order to exploit its full potential in agriculture.
Author contributions
SY, NSR, AS - Research concept and design; SY, NSR, HVL - Collection and/or assembly of data; SY, NSR, VS, SS, HVL, SM, SK, AS - Data analysis and interpretation; SY, NSR, VS, SS, HVL, JT, SM, SS, SK, AS - Writing the article; SY, NSR, VS, SS, SK, AS - Critical revision of the article; SY, NSR, AS - Final approval of the article.