Introduction
A homotetramer, ATP Citrate Lyase (ACLY), is a metabolic enzyme in charge of catalysing the glycolytic to lipidic metabolism, and it is highly expressed in adipose tissue and liver (Liang et al., 2023). By continuously hydrolysing adenosine triphosphate (ATP) to adenosine diphosphate (ADP) and phosphate (pi), ACLY produces acetyl CoA and oxaloacetate (OAA) from citrate and CoA. Acetyl CoA is essential in epigenetics, signalling, and cell metabolism. It serves as a precursor for the fatty acid synthesis pathway, mevalonate pathway, and acetylation reactions (Chypre, Zaidi, & Smans, 2012). It functions as a cofactor in histone acetylation, which is accountable for regulating gene expression and other proteins and RNA to regulate various biological activities. It also plays a vital role in energy generation (Zhao et al., 2016). Excessive expression of ACLY is implicated in the onset of numerous diseases. Its inhibition has spurred increased exploration for innovative inhibitors to address various conditions, including hypertriglyceridemia, obesity, chronic inflammation, type 2 diabetes, non-alcoholic fatty liver disease (NAFLD), cancer and metabolic syndromes (Feng, Zhang, Xu, & Shen, 2020; Lau & Giugliano, 2023; Wei et al., 2023). With its central role in cellular metabolism and association with disease states, ACLY has emerged as a potential therapeutic target. Various inhibitors designed to modulate ACLY activity are being explored for their efficacy in treating the associated conditions (Granchi, 2022). Among these inhibitors, Bempedoic acid is a pioneering, pro-drug-derived ACLY inhibitor that competitively hinders ACLY’s activity. This inhibition, in turn, modulates lipid metabolism by enhancing the expression and function of the hepatic low-density lipoprotein receptor LDLr (Feng et al., 2020). ACLY exhibits tissue-specific functions, with prominent roles identified in the liver that are essential for maintaining lipid homeostasis and preventing conditions such as hepatic steatosis.
In contrast, despite expressing ACLY, adipose tissue does not exhibit an overt phenotype. It shows that the lipid metabolism ought to be unaffected significantly by the presence or lack of ACLY in adipocytes. These disparate outcomes highlight the tissue-specific intricacies of ACLY function and its differential impact on lipid homeostasis in the liver versus fatty tissue. (Feng et al., 2020; Zhao et al., 2016).
This review aims to offer a detailed account of the many roles played by ACLY, investigating its complicated molecular process, structure, regulatory pathways, and involvement in various physiological and pathological conditions. It thoroughly analyses ACLY inhibitors, clarifying their molecular mechanism in clinical studies. The review concludes with a complete examination of the challenges involved in developing and optimising ACLY inhibitors, proposing potential strategies for conquering the challenges. It offers an exciting idea on possible routes for future ACLY inhibitor development, creating opportunities for advancements in understanding and therapeutic implications.
Biochemistry and Molecular Biology of ACLY
Location
ACLY is overexpressed in white adipose tissue, liver (Wang et al., 2010), cholinergic neurons and lactating mammary gland; it has low expression level in the heart, muscles, brain, and small intestine; ACLY activity is detected in pancreatic beta cells for the insulin secretion (Chu et al., 2010). In mammalian cells, the endoplasmic reticulum is bound by an extramitochondrial enzyme ACLY, generally called a cytosolic enzyme. Still, it also presents in nuclei of some mammalian cells, including human glioblastoma cells, colon carcinoma cells, mouse embryonic fibroblast and murine pro-B-cell lymphoid cells (Liang et al., 2023).
Structure
With 1101 amino acid residues, four identical subunits make up the homotetramer of human ACLY; each subunit is comprised of both a C-terminal citrulline-CoA lyase (CCL) module and an N-terminal citrulline CoA synthase (CCS) module, and ACLY has a total structure weight of 480 kDa (Shen, Wang, Tang, Zhu, & Wang, 2023). The configuration of ACLY contains five domains; the arrangement of ACLY domains starting at the peptide chain’s N-terminal is 3,4,5,1,2, and the CS domain. Domains 1 and 2 are present in the α-subunit and 3-5 are in the β-subunit, where domains 3 and 4 are ATP-grasp fold for the binding of ATP, domain 5 is the citrate binding site, the potential site of CoA binding corresponds to domain 1, the binding site of catalytically phosphorylated His760 is located in domain 2, and the CS domain is the central core of ACLY and it has sequence similarities with citrate synthase (Figure 1) (Icard et al., 2020; Sun, Hayakawa, Bateman, & Fraser, 2010; Wei, Schultz, Bazilevsky, Vogt, & Marmorstein, 2020). The C-terminal segment converts citryl-CoA to acetyl-CoA and oxaloacetate (Khwairakpam et al., 2020). Residues susceptible to phosphorylation on three serine or threonine residues are in the region between domain 5 and 1. Upon binding to ACLY, citrate forms hydrogen bonds between its hydroxyl and carboxyl group and a loop comprising residue 343-348. Additionally, Arg379 establishes a salt bridge containing citrate’s pro-R carboxylate (Zu et al., 2012).
Reaction
ACLY promotes the transformation of citrate and CoA to OAA and acetyl CoA through the concurrent hydrolysis of ATP to ADP and Pi. The catalytic reaction of ACLY is based on three different consecutive steps. a) It was initiated by the phosphorylation of His760 with Mg-ATP to Mg-ADP, generating an unstable citryl-phosphate. b) The thiol group of CoA attacks the enzyme bounded citryl-phosphate to form the citryl-CoA and Pi. c) Finally, through the retro-Clasen reaction, the enzyme-bound intermediate citral-CoA undergoes cleavage into acetyl CoA and OAA, both of which are subsequently released from the enzyme’s active site (Figure 1) (Dominguez, Brüne, & Namgaladze, 2021; Elshourbagy et al., 1992; Fan et al., 2012; Granchi, 2018; Wei et al., 2019).
Mechanism
Acetyl CoA is a versatile biomolecule that participates in biochemical reactions such as protein, carbohydrate, and lipid metabolism (Dominguez et al., 2021). Acetyl CoA is produced in mitochondria and is a significant precursor for synthesising lipids and acetylation of histones. In the process of glycolysis, glucose is converted into pyruvate. Subsequently, pyruvate undergoes oxidative decarboxylation facilitated by the mitochondrial pyruvate dehydrogenase complex (PDC), generating acetyl-CoA. It cannot traverse the mitochondrial membrane to the cytoplasm, where lipid biosynthesis occurs. Therefore, within the mitochondria, citrate synthase mediates the synthesis of the acetyl-CoA with OAA, resulting in the formation of citrate. Citrate transporter in the mitochondrial membrane transports the citrate from mitochondria to the cytosol, where the cytosolic enzyme ACLY cleaves citrate, regenerating acetyl CoA and oxaloacetate, and it serves as a central hub in cellular metabolism, connecting various metabolic pathways. Alternatively, citrate can undergo oxidation via the tricarboxylic acid (TCA) cycle ( ; Shi & BP Tu., 2015) (Bose, Ramesh, & Locasale, 2019; Izzo et al., 2023; Zhao et al., 2016).
Regulation
The ACLY gene is regulated at multiple levels. Its promoter contains a sterol response element (SRE), controlled by sterol regulatory element binding protein1α (SREBP-1α), and ACLY undergoes Akt-dependent phosphorylation (Ser454), contributing to stabilisation. Nucleoside diphosphate kinase and cyclic adenosine monophosphate (cAMP)-dependent protein kinase also phosphorylate ACLY. This phosphorylation regulates nuclear acetylation, influencing inflammation and deoxyribonucleic acid (DNA) damage repair in various cell types. Pathological factors, such as acute myocardial infarction and obesity-related insulin resistance, can elevate ACLY expression. Epigenetically, KDM5B upregulation in breast cancer promotes ACLY expression. Still, its knockdown, as seen in Michigan cancer foundation-7 (MCF-7) and MDA-MB-231 cells, decreases ACLY by acting AMP-activated protein kinase (AMPK) (Feng et al., 2020; Sato et al., 2000; Zhang et al., 2019).
Immunity
An essential enzyme in macrophage inflammation, ACLY reacts to stimuli such as TNFα, IL-4, LPS, and IFNγ. In M1 macrophages, STAT and NF-KB transcription factors regulate ACLY in response to LPS, while in M2 macrophages, IL-4 signalling activates ACLY via the Akt-mTORC1 pathway. M2 gene induction and increased histone acetylation are caused by ACLY activity. The production of inflammatory mediators (ROS, PGE2, and NO) by M1 macrophages depends on ACLY, and when ACLY is inhibited, these mediators are significantly reduced, impacting immune cell recruitment, inflammation, and vasodilation. Increasing ACLY activity benefits the host’s defence against pathogen invasion (Covarrubias et al., 2016; He et al., 2017; Infantino, Iacobazzi, Palmieri, & Menga, 2013).
Pathways
One major pathway utilising ACLY is fatty acid synthesis. Fatty acids (FAs) have diverse roles in cells, functioning as essential structural elements in cell membranes to energy providers and contributors to the synthesis of signalling molecules (Carvalho & Caramujo, 2018). They are the foundational components for various lipid types, including phospholipids, sphingolipids, and triglycerides (Li, Zhang, Qiu, Deng, & Wang, 2022). Fatty acid synthesis is carried out by a metabolic process called de novo lipogenesis (DNL). ACLY is the initial crucial enzyme in this pathway, converting citrate to acetyl-CoA, an essential building block for synthesising fatty acids. Acetyl-CoA is converted to malonyl-CoA, catalysed by acetyl-CoA carboxylase (ACC). Subsequently, fatty acid synthase (FASN) catalyses the condensation of malonyl-CoA with acetyl-CoA, is an irreversible carboxylation, and generates FA 16:0, saturated 16-carbon FA palmitate. Following the desaturation process of palmitate, monounsaturated fatty acids via stearoyl-CoA desaturase (SCD), featuring a double bond at position ∆9. Simultaneously, the elongation, facilitated by the fatty acid elongases like ELOVL6, involves the addition of two-carbon units to palmitate, ultimately resulting in the formation of the saturated fatty acid stearate (Figure 3) (Koundouros & Poulogiannis, 2020).
The mevalonate pathway is an essential biochemical pathway involved in synthesising sterol and non-sterol isoprenoids, which are vital in multicellular functions (Buhaescu & Izzedine, 2007). The path begins with the molecule acetyl-CoA. It was transformed into mevalonate accelerated by the enzyme 3-hydroxy-3-methylglutaryl coenzyme A reductase (HMGCR) through a series of chemical reactions. Similarly, mevalonate converts to farnesyl pyrophosphate (FPP), a critical intermediate in many pathways, including geranylgeranyl pyrophosphate (GG-PP) and cholesterol biosynthesis. Farnesylation and geranylgeranylation are processes that involve FPP and GG-PP, collectively referred to as prenylation, a process where they are attached to Ras and Rho family proteins and influence their functions (Figure 3) (Tricarico, Crovella, & Celsi, 2015; Zaidi, Royaux, Swinnen, & Smans, 2012; Zaidi et al., 2012).
Furthermore, ACLY significantly functions in acetylation reactions by supplying acetyl-CoA, which modifies proteins (Zaidi et al., 2012). Protein acetylation significantly influences human protein’s localisation, stability, expression, and function (He et al., 2017). In mammalian cells, its enzymatic activity frequently depends on the Akt pathway. In histone acetylation, the acetyl group is added to a specific lysine residue on a histone protein, resulting in the altering of chromatin structure, affecting gene expression, epigenetic regulation of macrophage activation and cellular processes (Figure 3) (Granchi, 2018; Guo et al., 2020; Sivanand et al., 2017; Zaidi et al., 2012).
Pathophysiological role of ACLY
ACLY itself is not directly associated with specific disorders, but dysregulation of its activity can contribute to various health conditions, including:
Cancer
One of the biggest threats to global public health is cancer, which stands as the second most prevalent cause of mortality (Icard, Wu, Alifano, & Fournel, 2019; Siegel, Miller, Wagle, & Jemal, 2023). The function of ACLY is vital in cancer by channelling excess glycolytic activity into lipid synthesis, promoting essential metabolic changes crucial for tumor growth and differentiation. The interplay between ACLY overexpression and the integrin ITGA2, IGF-1, and beta-catenin signalling pathways appears to create a conducive environment for the onset and course of cancer models by promoting both stemness and metastasis (Martin-Perez, Urdiroz-Urricelqui, Bigas, & Benitah, 2022). Distinctive increase in ACLY activity and expression has been reported in liver, breast, stomach, bladder, prostate, lungs, and colon tumors. Thus, increased expression of ACLY in various cancer cell types, coupled with the observation that inhibiting ACLY leads to a halt in cancer cell proliferation both in vivo as well as in vitro, suggests the significant role of the enzyme in driving the progression of cancer cells (Figure 4) (Icard et al., 2019; Zaidi et al., 2012).
Cardiovascular disease
Currently, the world’s leading cause of death is cardiovascular disease (CVD). Type 2 diabetes mellitus, dyslipidemia, sex, age, hypertension, obesity, and metabolic syndrome are major risk factors for CVD, which induce vascular inflammation and endothelial dysfunction, resulting in atherosclerosis (Qin et al., 2017; Srikanth & Deedwania, 2016). Atherosclerotic cardiovascular disease (ASCVD), consisting of myocardial infarction and ischemic stroke, stands as the predominant cause of illness and death worldwide (Sandesara, Virani, Fazio, & Shapiro, 2019). ACLY is linked to cardiovascular disease through its involvement in lipid synthesis. Overexpression of ACLY leads to a rise in the production of fatty acids and cholesterol that cause plaques to build up in blood vessels, contributing to one of the leading causes of cardiovascular disease, atherosclerosis. These plaques can narrow the blood vessels, reducing the blood flow and causing complications such as stroke and heart attacks (Linton et al., 2019; Verberk, Kuiper, Lauterbach, Latz, & Bossche, 2021). The results of Mendelian randomisation studies have affirmed that ACLY is a therapeutic target for minimising low-density lipoprotein (LDL-C) levels and promoting cardiovascular well-being (Feng et al., 2020; Ference et al., 2019). Inhibiting ACLY is proposed to positively impact atherosclerosis by increasing the activity of sterol transporter ATP-binding cassette transporter G5/8(ABCG5/8), essential in overseeing reverse cholesterol transport’s ultimate phase. This process facilitates the movement of cholesterol to the hepatobiliary system. Thus, ACLY inhibition exhibits an anti-atherosclerotic effect by increasing the expression of ABCG5/8, potentially promoting the removal of cholesterol and contributing to atherosclerosis prevention (Figure 4) (Feng et al., 2020).
NAFLD
Non-Alcoholic Fatty Liver Disease (NAFLD) comprises a range of liver issues, from primary fat accumulation to more severe conditions like steatohepatitis, cirrhosis, and potentially liver cancer. It is closely linked to obesity, abnormal lipid levels, insulin resistance, and high blood pressure, essentially portraying the liver-related aspects of metabolic syndrome. The liver experiences a reduction in SIRT2 expression following overnutrition, causing an increase in ACLY’s acetylation of ACLY at Lys-554, Lys-546, and Lys-540 (ACLY 3K). This heightened acetylation of ACLY-3K increases protein stability, thereby fostering de novo lipogenesis. Consequently, this phenomenon aids in developing hepatic steatosis and could be involved in the pathological advancement of NAFLD (Figure 4) (Guo et al., 2019).
Neurodegenerative disease
Alzheimer’s disease (AD) is a relentless and progressive neurodegenerative condition that impacts vast areas of the hippocampus and cerebral cortex (Masters, Bateman, & Blennow, 2015). Behavioural issues, as well as memory and cognitive impairment, are signs of AD. ACLY has been positioned in cholinergic nerve terminals. In neuronal tissue, ACLY plays a crucial role in the synthesis of acetyl CoA, a necessary precursor for the production of acetylcholine (Khwairakpam et al., 2020). Acetylcholine is a chemical that serves as a neurotransmitter that carries messages through nerve cells from brain to body, which contributes to functions such as memory, learning (Hasselmo, 2006), arousal, motivation, and attention (Sam & Bordoni, 2022). The presence of ACLY in cholinergic nerve terminals and its elevated beta-galactosidase expression suggest that ACLY is necessary for the synthesis of acetylcholine. The brain tissue of individuals with AD exhibits a reduction in ACLY activity. One of PHP’s known substrates is ACLY, which is reduced by dephosphorylation. SN56 cholinergic neuroblastoma cells showed a significant increase in acetylcholine levels upon reduction of PHP. These findings suggest that the reduced ACLY activity may contribute to Alzheimer’s disease. Upregulation of ACLY was found in Batten disease, and it correlated with the disease's high levels of oxidative stress. Overall, the findings indicate that the dysregulation of ACLY is one of the reasons for the development of neurodegenerative disease (Khwairakpam et al., 2020). Familial Dysautonomia (FD) is a peripheral neuropathy with an autosomal recessive inheritance pattern that profoundly impairs the levels of the expression of Elp1 in the nervous system of patents with FD. The restoration of both Tau levels and neurite morphology through ACLY overexpression suggests that ACLY’s activity plays a regulatory role in the mechanism responsible for Tau-mediated abnormalities in neurite morphology observed in Elp1 knockdown, suggesting a potential link between dysfunction in both Tau and ACLY in the pathophysiology of FD as well as other neurodegenerative conditions marked by compromised axonal transport and Tau malfunction (Figure 4) (Shilian, Even, Gast, Nguyen, & Weil, 2022).
ACLY Inhibitors: Development and Mechanism of Action
ACLY inhibitors are compounds that can block the activity of ACLY. Research and drug development efforts have identified several compounds as potential ACLY inhibitors. Some of the significant inhibitors are listed in Table 1 and have been detailed below:
Table 1
A list of different ACLY inhibitors with their IC50 value.
ACLY Inhibitors | Source | Mechanism of action | Potency | Assay | Reference |
---|---|---|---|---|---|
(-)-hydroxycitric acid | Garcinia atroviridis, Garcinia indica, Garcinia cambogia (fruit rinds) | lowers FA synthesis, blood cholesterol, and obesity | G. atroviridis IC50 10µ for MCF7 and 70µM for LCC2. G. indica IC50 = 11.34mM. G. cambogia IC50 value for LN229 = 12.1 mM and U87 = 16.7mM | SRB, Proliferation assay, Cell migration assay | |
Bempedoic acid | Prodrug | Decreases cholesterol synthesis in the liver, LDL-C, apo B, and reduce myotoxicity | IC50: 3.17 µg/mL; IC50 value for cholesterol = 9.7 µm, cholesteryl ester = 8.4 µm, and triglycerides = 17.8 µm. IC50 against SARS-CoV-2 - WT = 14.4 µM Delta = 6.3µM Omicron = 7.8 µM. IC50 = > 1000 µM | Population pharmacokinetics/ pharmacodynamics PopPK/PD analysis; ACL enzyme activity assay; qRT-PCR; hERG assay | Jadhav et al. (2023)Pinkosky et al. (2016) (Yuen et al., 2022) Amore, Cramer, Macdougall, Sasiela, and Emery (2021) |
326E | Small molecule | Reduction in lipogenesis and ameliorates dyslipidemia | IC50 =5.31±1.2µmol/L | ADP-Glo assay | |
Curcumin | Naturally occurring polyphenolic compound | Reduces hepatic lipid deposition, hyperlipidemia, and body weight | - | - | |
SB-204990 | Synthetic compound | lowers lipogenesis metabolic effects and exhibits antitumor properties | IC50 against SARS-CoV-2 - WT = 15.7µM Delta = 13.1µM Omicron = 11.7 µM; IC50 values for A549 = 24 µM; PC3 = 26 µM; SKOV3 = 53 µM | qRT-PCR; In vitro antitumor sensitivity assay | |
BMS-303141 | Synthetic compound | suppress osteoclast formation, induce apoptosis in HCC cells, prevent bone loss, reduce weight gain | IC50 = 0.13 µM; IC50 = 0.60 ± 0.72 µmol. L-1; IC50 = 0.94 µM | Enzyme inhibition assay; ADP-Glo assay; ACL-MDH Coupled assay | Zhang, Hu, Qiu, Li, and Bian (2023)Zhou et al. (2023)Ma, Chu, and Cheng (2009) |
2-Furoic acid | - | Lowers serum triglycerides and cholesterol levels and increases HDL-C | - | - | |
MEDICA 16 | Derivative of dicarboxylic acid | Effect on lipid synthesis and insulin sensitisation | Ki value = 16 µM | ACLY inhibition assay | |
Baker’s yeast glucan (BYG) | Polysaccharide | Down-regulate lipid biosynthesis | - | - | |
Cucurbitacin B | Bioactive compound in cucumber | Anti-cancer effect | IC50 = (~ 0.3 µM for PC-3 and LNCaP) | CellTiter-Glo®luminescent cell viability assays | |
Resveratrol | Polyphenolic flavonoid | Prevents dyslipidemia | - | - | |
Cinnamon polyphenol | Extract from Cinnamon | Low oxidative stress suppresses hepatic ACLY expression. | - | - | |
3-Thiadicarboxylic acid | Sulfur-substituted fatty acid analogue | Inhibits FA synthesis | - | - | |
NDI-091143 | Allosteric inhibitor | competitively inhibits citrate substrate | Ki value = 7.0 nM; IC50 = 2.1 nM; IC50 = 44.0 ± 3.0 nM | ADP-Glo enzymatic assay; MDH coupled enzyme assay | |
Forrestiacids J & K | triterpene-diterpene hybrids | Management of ACLY-related disease | IC50 = 1.8 to 11µM | Enzyme inhibition assay | |
2-chloro-1, 3, 8-trihydroxy-6-methyl-9-anthrone | Metabolite of microorganisms | Hypolipidemic agent | IC50 = 283nM; Ki of <100nM | - | |
Antimycins | Produced by Streptomyces species | inhibitory activity against ACLY | Ki value of - A2= 4.2 µM ; A8= 4.0 µM | Enzymatic assay | |
10, 11-dehydrocurvularin | Organic macrolide derived from fungi | Anti-cancer effect | IC50 = 0.93 µM | ADP-Glo enzymatic assay | |
Furan carboxylic derivatives | Furan carboxylate moiety (fused compound) | Anti-cancer effect | IC50 value - for A1= 4.1 µM; A3= 11.9 µM; C4=13.8 µM | ADP-Glo enzymatic assay | |
Radicicol | Macrolide isolated from Monosporium bonoredn | Block insulin secretion stimulated by glucose | Ki = 13 µM for citrate and 7µM for ATP | Enzymatic assay | |
(+)-2,2-difluorocitrate | Enantiomers | Irreversibly inactivates aconitase | Kis = 0.7 µM | Enzyme inhibition assay | |
Crocins I ana II | Natural compound, isolated from Gardenia jasminoides | - | IC50 values for Crocin I = 36.3 ± 6.24 Crocin II = 29.7 ± 7.41 µM | Enzyme inhibitory assay | |
Purpurone | non-crystalline glassy solid | hinders the synthesis of FAs | IC50 = 7 µM | Enzymatic assay | |
Alkenyl diacids | Derivative of alkenyl diacids | demonstrated increased antitumor cell proliferation effects | IC50 = 1.5 µmol/L | Enzyme inhibition assay | |
Formyl phloroglucinol meroterpenoids | Natural compound, isolated from Eucalyptus globulus subsp. Maidenii | Lowers hepatocyte lipogenesis | - | - |
4.1. (-)-hydroxycitric acid
The (-)-hydroxycitric acid, also known as (2S,3S)-2-hydroxycitrate, was the first discovered natural ACLY inhibitor (Sullivan, Singh, Srere, & Glusker, 1977) was isolated from fruit rinds of Garcinia atroviridis, Garcinia indica, and Garcinia cambogia (Hu, Komakula, & Fraser, 2017; Jena, Jayaprakasha, Singh, & Sakariah, 2002; Lewis & Neelakantan, 1965). Hydroxycitric acid (HCA) treatment lowers blood cholesterol, obesity, and fatty acid synthesis (Roy et al., 2003). HCA lowers lipogenesis and biosynthesis of fatty acids, which result from reducing acetyl-CoA units by blocking ACLY (Uddandrao et al., 2023). In addition, HCA seems to upregulate the genes encoding serotonin receptors and raise endogenous serotonin concentrations. These actions promote oxidation of fullness fat and lower de novo lipogenesis (Baroni et al., 2020).
Preclinical studies
A previous study explores the potential synergistic effects of combining Hydroxycitric Acid (HCA) with Tamoxifen (TAM), a treatment that is regularly utilised for estrogen receptor (ER)-positive breast cancer. The researchers hypothesise that focusing on lipid metabolic enzymes may improve TAM’s ability to kill breast cancer cells. A study using the MCF-7 ER-positive cell lines for breast cancer shows that TAM and HCA co-treatment prompt apoptosis, reduce cell viability, and decrease expression of ACLY, acetyl CoA carboxylase alpha (ACC-α), and fatty acid synthase (FAS) in a synergistic manner. This study indicates that the HCA-induced inhibition of ACLY may improve TAM’s therapeutic efficacy against breast cancer (Ismail et al., 2022; Ismail, Doghish, Elsadek, Salama, & Mariee, 2020). Another study states that, in a 28-day trial, broiler chickens fed a diet containing (-)-HCA significantly reduced body weight and triglycerides (TG) content and increased hepatic glycogen. Molecular analysis revealed decreased expression of lipogenic genes (SREBP-1c, ACLY, FAS) and increased expression of lipolysis-related genes (AMPKβ2, PPARα) IN (-)-HCA supplemented chickens compared to controls. This suggests that (-)- HCA effectively hinders fat production and enhances fat breakdown, decreasing abdominal fat deposition in broiler chickens (Han, Li, Wang, & Ma, 2016).
Clinical trials
In an open-label clinical study involving 100 obese individuals aged 20-45, participants were given a single Garcinia caplet containing 350 mg powder and 250 mg extract (60-66% HCA) twice daily for three months. The study significantly reduced serum triglyceride, LDL, high-density lipoprotein (HDL), cholesterol levels, triceps, subscapular, body weight, and mid-axillary measurements after HCA dosage. According to these results, HCA may be a valuable intervention for managing obesity (Tomar et al., 2019).
Bempedoic acid
Bempedoic acid (8-hydroxy-2,2,14,14-tetramethylpentadecaned–ioic acid; ETC-1002) is a prodrug. Therefore, the enzyme (ACSVL1), very long-chain acyl-CoA synthase-1, catalyses the conversion of bempedoic acid in its active form, bempedoyl CoA, inhibiting ACLY and activating AMPK. ETC-1002 is orally administrated with a half-life of 15-24h (Bilen & Ballantyne, 2016; Biolo et al., 2022); the USA and European Union approved it for patients who are intolerant to statins (Ballantyne et al., 2021). Because the ACSVL1 enzyme is primarily expressed in the kidney and liver rather than in skeletal muscles or other tissues, bempedoic acid activity is essentially limited to the liver; it reduces myotoxicity compared to statins. ACLY inhibition inhibits a cholesterol synthesis pathway in the liver that induces upregulation of LDL receptors, lowering LDL-C in the liver (Gupta et al., 2020; Tummala et al., 2022). In patients with hypercholesterolemia or mixed dyslipidemia, bempedoic acid lowers apo B (15%), non-HDL-C (19%), LDL-C (20-25%) and total cholesterol (16%; ) (Ferri, Ruscica, Fazio, & Corsini, 2024). Bempedoic acid is primarily prescribed to patients suffering from heterozygous familial hyper-cholesterolemia (HeFH) and atherosclerotic cardiovascular disease (ASCVD) (Brandts & Ray, 2020). ETC-1002–CoA has very few adverse effects, including hyperuricemia due to the major metabolite of bempedoic acid; glucuronide serves as an OAT (organic anion transporter) substrate (Roth, Obaidat, & Hagenbuch, 2012), resulting in the competition between glucuronide and uric acid for the same carrier OAT2 transporter (Jialal & Ramakrishnan, 2022). Some rare AEs are prostatic hyperplasia, atrial fibrillation, and decreased haemoglobin levels (Ballantyne et al., 2021; Bays et al., 2020). Bempedoyl CoA also inhibits the rate-limiting enzymes HMG-CoA reductase and acetyl-CoA carboxylase (Filippov, Pinkosky, & Newton, 2014) non-high-density lipoprotein cholesterol and apolipoprotein B (Lau & Giugliano, 2023). Bempedoic acid alone is effective, but combined with other drugs like statins and ezetimibe, it significantly reduces LDL-C. Statins are intolerant to patients due to myalgia; therefore, patients can take bempedoic acid as an alternative treatment option for those who are unwilling or unable to take statins. Bempedoic acid also has more significant benefits than placebo (Bilen & Ballantyne, 2016).
Preclinical studies
ETC-1002 (for 12 weeks, from 3 to 30 mg/kg body weight per day) significantly decreased elevated cholesterol (up to 64%) and enhanced glucose tolerance in Ldlr-/- mice fed a high-fat, high-cholesterol diet. In a study using LDL receptor-deficient Yucatan miniature pigs fed a diet heavy in cholesterol and given bempedoic acid orally, heterozygous pigs showed a notable decrease in total cholesterol (41%) and LDL-cholesterol (61%) with a human-equivalent dose of 120 mg/day. Homozygous pigs showed lesser reductions (LDL-cholesterol was 29% and total cholesterol was 27%, respectively) and no notable impact on glucose, insulin, HDL-cholesterol, and triglycerides. Both heterozygous and homozygous pigs experienced substantial reductions in aortic and coronary lesions, indicating the drug’s efficacy in mitigating atherosclerotic lesions (Ruscica, Banach, Sahebkar, Corsini, & Sirtori, 2019).
Clinical trials
In a phase 2 clinical trial involving 60 individuals with both hyperlipidemic patients with type 2 diabetes, who had stopped taking medications for diabetes and lipid control, were randomly assigned and placed into either the bempedoic acid group (receiving 80 mg once daily for two first two weeks, followed by 120mg once daily for next two weeks) or the placebo group. The result revealed a notable decrease in LDL-C, total cholesterol, hsCPR, and non-HDL-C. Additionally, the patients exhibit good tolerance to bempedoic acid (Feng et al., 2020; Gutierrez et al., 2014). Another research study enrolled 2,230 patients (with or without HeFH and ASCVD) and administered statin therapy at the highest tolerated dose. A sum of 1488 patients was assigned to undergo treatment with bempedoic acid (administrated at a daily dose of 180 mg, once per day, over 52 weeks), while 742 individuals were designated to receive a placebo. The findings revealed no notable distinction in adverse events between the two groups (Ray et al., 2019). In previous research across seven phase 2 trials that randomised 977 participants, with 699 individuals receiving the active drug, ETC-1002 exhibited the ability to lower LDL-C levels. This reduction occurred when ETC-1002 was administered as a standalone treatment, in conjunction with ezetimibe, and as an augmentation to statin therapy. The most significant decrease in LDL-C levels was noted in individuals with a history of statin intolerance when ETC-1002 was employed with ezetimibe (Bilen & Ballantyne, 2016).
Safety of bempedoic acid in hyperlipidemia
In a comprehensive analysis of bempedoic acid’s safety involving 3621 patients across four phase-three randomised-controlled trials, patients received 180mg of bempedoic acid or placebo. They maximally tolerated statin therapy for a median of 363 days. The rates of treatment-emergent adverse events were 87.1 per 100 person-years for individuals receiving bempedoic acid and 82.9 per 100 person-years for those on the placebo. Interestingly, the occurrence of reported muscle symptoms was comparable in both groups. Bempedoic acid demonstrated a lower frequency of new-onset diabetes or hyperglycemia, with rates of 4.7 per 100 patient-years compared to 6.4 per 100 patient-years for the placebo. Some biochemical markers showed small changes with no clinical relevance, and gout incidence was higher with bempedoic acid but reversible upon therapy cessation. The increased gout risk is likely linked to the drug’s reversible impact on the kidney’s OACT2 transporter and does not indicate toxicity (Banach et al., 2023; Bays et al., 2020; Nissen et al., 2023). A modest increase in aminotransferase elevations (exceeding three times the upper threshold of the normal range) is associated with bempedoic acid at a rate of -0.8/100 patient-years, as opposed to a rate of 0.3 per 100 patient-years for the placebo group. This effect is temporary and can be reversed when treatment is stopped. While tendon rupture has been linked to a heightened risk in bempedoic acid therapy, this risk has only been assessed in two trials (CLEAR Harmony and CLEAR Wisdom) involving ten patients who also showed additional risk factors. More post-marketing surveillance is needed to fully comprehend the significance of these observations (Bays et al., 2020). In the CLEAR outcomes study, the bempedoic acid and placebo groups displayed similar overall occurrences of adverse events, serious adverse events, and instances leading to discontinuation. Only slight variations were observed between the groups for pre-specified adverse events of particular interest, such as elevated hepatic enzyme levels and renal events. The placebo group reported more cases of myalgias, whereas the bempedoic acid group had slightly higher incidences of cholelithiasis, gout, and hyperuricemia. Bempedoic acid seems generally safe, well-tolerated, and linked to minor side effects that are easily remedied (Banach et al., 2023; Nissen et al., 2023; Raschi et al., 2023).
326E
A new ACLY inhibitor, 326E is a low molecular-weight compound featuring an enedioic acid structural component, and its CoA-conjugated version, 326E-CoA, acts as an inhibitor of ACLY activity with an IC50 value of 5.31±1.2µmol/L. The small molecule 326E is an isomer of 326F, which is a simple dehydrated product of ETC-1002. Compared with bempedoic acid, 326E was quickly absorbed following oral delivery and exhibited a high blood exposure. 326E effectively reduces lipogenesis and ameliorates dyslipidemia within a rodent model experiencing hyperlipidemia caused by a high-fat, high-cholesterol (HFHC) diet. Although the 326E inhibitor is under study, it has recently obtained approval for clinical trials targeting hyperlipidemia (Xie et al., 2023).
Preclinical studies
326E is also called BGT-002 (Liang et al., 2023). After BGT-002 is thoroughly metabolised, ZM326E-M2, a significant circulating ACLY monoglucuronide metabolite, is produced. The LC-MS/MS method was employed to quantify human plasma simultaneously. Pharmacokinetic analysis following a 100 mg oral dose revealed a delayed tmax (4.00 h) for ZM326E-M2, with 14.1% peak concentration and 19.5% plasma exposure compared to the parent drug BGT-002, which exhibited rapid absorption (tmax of 1.13 h). The study underscores the necessity of a thorough evaluation of the safety and efficacy of ZM326E-M2 in clinical research (Hu et al., 2024; Liang et al., 2023).
In hamster and rhesus monkeys, long-term 326E treatment led to a marked reduction in hyperlipidemia. For 24 weeks, oral administration of 326E once daily inhibited the development of atherosclerosis by inhibiting ACLY by enhancing the intestinal absorption of hepatic cholesterol and decreasing hepatic de novo lipogenesis in ApoE-/- mice more than treatment with bempedoic acid (Xie et al., 2023).
Curcumin
Curcumin is a naturally occurring polyphenolic compound in turmeric, used as a nutrient supplement or food additive, which can manage metabolic disorders like obesity, NAFLD, and type 2 diabetes. It also reduces the deposition of abdominal fat and markedly decreases the expression of ACLY (Maithilikarpagaselvi, Sridhar, Swaminathan, Sripradha, & Badhe, 2016; Xie et al., 2019).
Preclinical studies
Curcumin, a lipid-lowering drug, reduces hyperlipidemia and hepatic lipid deposition in mice fed a high-fat plus high-fructose diet (HFHFD) and oleic and palmitic acid (OPA). It might have prevented NAFLD by modifying the expression and functionality of ACLY and SLC13A5, confirming its potential as a preventive measure against NAFLD (Sun et al., 2021). A prior study examined the effect of curcumin on lipid metabolism in the livers of broiler chickens. Results showed that curcumin significantly decreased body weight, hepatic lipid profile and weight of abdominal fat. Curcumin down regulates the genes linked to lipolysis and lipogenesis, such as ACLY, CPT-1, PPARα, FAS, ACC, and SREBP-1c (Xie et al., 2019). In another research, four groups of forty male Wistar rats each were given a standard rodent diet, while the other two groups received a diet high in fructose (60%) for ten weeks. Each diet was given concurrently with an administration of curcumin (200 mg/kg body weight). Fructose-fed rats supplemented with curcumin had improved lipid profiles and decreased adipose, body, and liver weight. Notably, curcumin raised HDL-C and reduced plasma levels of VLDL-C, triglycerides, and lipid ratios by inhibiting ACLY (95%), FAS (77%), and ACC (50%), preventing hyperlipidemia and hepatic steatosis caused by high-fructose diet in rats (Maithilikarpagaselvi et al., 2016).
SB-204990
SB-204990 has been employed in previous research studies as an ACLY inhibitor prodrug (an active form converted into an active drug) of SB-201076 (Pearce et al., 1998). In rat hepatocytes and HepG2 cells, SB204990 suppresses lipogenesis and fatty acid synthesis (Vlijmenº et al., 1998). Elevated platelet activity is a common outcome of diabetes, with the activated platelets notably causing a substantial release of mitochondrial acetyl CoA. SB204990 diminishes acetyl-CoA levels in platelet cytoplasm, concurrently impeding malondialdehyde (MDA) formation and the aggregation of platelets. This implies that targeting ACLY could also potentially mitigate platelet hyperactivation (Michno, Skibowska, Raszeja-Specht, Ćwikowska, & Szutowicz, 2004).
Preclinical studies
Following a high-fat diet, the ACLY inhibitor SB-204990 was administrated to wild-type mice and showed beneficial effects on physical strength and metabolic health. However, in mice on a healthy diet, it led to mild insulin resistance and metabolic imbalance. Utilising the multi-omics approach, the research findings indicate that SB-204990 controls molecular pathways associated with aging, including the folate cycle, mitochondrial function, mTOR signalling, and energy metabolism. These findings suggest a potential therapeutic strategy to prevent metabolic abnormalities associated with an unhealthy diet and aging (Sola-García et al., 2023).
BMS-303141
BMS-303141 emerges as a promising therapeutic target for suppressing ACLY. The presence of ACLY in hepatocellular carcinoma (HCC) cells promotes these cell’s proliferation, migration, and invasion. In contrast, the ACLY inhibitor BMS-303141 induces apoptosis in HCC cells by instigating endoplasmic reticulum (ER) stress, which promises to augment the effectiveness of HCC treatment when combined with sorafenib. However, several limitations are inherent in this study (Zheng et al., 2021). BMS-303141 exhibits a substantial reduction of nucleo-cytosolic acetyl-CoA in both osteoclasts and BMMs, leading to the inhibition of osteoclast formation in vitro (Guo et al., 2020). Moreover, ACLY inhibitor BMS-303140 protects against renal injuries associated with obesity (Zhan et al., 2022).
Preclinical studies
The ACLY inhibitor BMS-303141 demonstrated a significant reduction in nucleo-cytosolic acetyl-CoA levels in both osteoclasts and Bone Marrow-Derived Macrophages (BMMs), leading to the suppression of osteoclast formation in vitro. In a high-fat diet mouse model, BMS-303141 exhibited the ability to inhibit ACLY with oral dosages ranging from 10 to 100 mg/kg/d. The results showed that BMS-303141 effectively suppressed osteoclast formation, prevented bone loss induced by ovariectomy (OVX) and significantly decreased the acetylation level of histone H3 during osteoclast differentiation (Guo et al., 2020). In a prior study, BMS-303141 was orally administered to 12-week-old db/db mice over 30 days. It exhibited the highest binding affinity for ACLY and reduced ACLY expression within the kidneys of db/db mice. It indicates that the administration of BMS-303141 resulted in decreased serum lipid levels and downregulated renal lipogenic enzymes, including HMGCR, ACC, and FAS, while also attenuating renal esterified linoleic acid (ELA) levels in db/db mice. Reducing ELA improved tubulointerstitial fibrosis, injuries, and inflammations (Zhan et al., 2022).
2-Furoic acid
2-Furoic acid has demonstrated encouraging results as a possible cholesterol-controlling agent. It effectively reduced serum cholesterol and triglyceride levels in rats while raising HDL cholesterol by inhibiting ACLY and other enzymes (Hall et al., 1993).
Preclinical studies
Using 20 mg/kg/day of 2-furoic acid orally in rats significantly reduced serum cholesterol and triglyceride levels and increased HDL cholesterol in preclinical trials. The compound showed elevated HDL receptor activity and decreased LDL receptor activity following intracellular enzyme interference. In vivo treatment decreased essential enzyme activities, including ACLY, in the small intestine and liver. On the other hand, elevated lithogenic bile salts raised concerns. With a comparatively low LD50 of 250 mg/kg intraperitoneally, acute toxicity studies in mice revealed some hepatic toxicity (Hall et al., 1993).
MEDICA 16
MEDICA 16, a derivative of dicarboxylic acid with β, βʹ-methyl substitution in an α, ω-dicarboxylic acid structure, has shown a strong ability to inhibit the synthesis of lipids in mouse livers. Because of its ability to lower cytosolic acetyl-CoA, this unique compound has a significant hypocholesterolemic effect. Except for a deliberate dimethyl substitution at position 3 of the carbon chain, MEDICA 16 behaves like a typical fatty acid, which was the basis for its structural similarity to long-chain fatty acids. Because of this modification inhibits β-oxidation, making MEDICA 16 an inactive fatty acid substitute (Bar-Tana et al., 1989).
Preclinical studies
In vivo tests demonstrated that MEDICA 16 effectively inhibited lipid synthesis in mice livers. The compound’s capacity to lower acetyl-CoA levels was associated with the notable hypocholesterolemic effect observed. Further in vitro research examined MEDICA 16’s mechanism of action. With a ki value of 16µM, it was discovered to competitively inhibit rat liver ACLY, decreasing the synthesis of FAs and cholesterol in cultured rat hepatocytes. The diacid form and the CoA-monothioester of MEDICA 16 both showed an inhibitory effect on ACC, which helped to explain the observed hypolipidemic impact, indicating that MEDICA 16’s action was not limited to ACLY (Bar-Tana et al., 1985; Bar-Tana et al., 1985; Granchi, 2018). Additionally, MEDICA 16 showed promise as a sensitiser, improving insulin responsiveness in liver, muscle, and adipose tissues in animal models of obesity-induced insulin resistance. Reduced insulin-induced hepatic glucose synthesis and increased insulin-induced total body glucose disposal indicated this. With its diverse effects on lipid metabolism, MEDICA 16 shows promise as a compound that may be used to manage hypocholesterolemia and improve insulin sensitivity in case of obesity-induced insulin resistance (Atkinson, Kelly, Russell, Bar-Tana, & Lopaschuk, 2002; Granchi, 2018).
Baker’s yeast glucan (BYG
Baker’s yeast glucan (BYG) is a type of polysaccharide that has gained attention for its anti-diabetic effects. It inhibits ACLY and other genes related to glycerolipid synthesis, gluconeogenesis, and lipid biosynthesis.
Preclinical study
In a study, BYG was administered in ob/ob mice as a model to assess the impact of BYG. Oral administration of BYG reduced hepatic disorder and blood glucose, up-regulating phosphorylated AMPK and AKT while down-regulating ACLY expression. RNA-Seq analysis revealed BYG’s impact on downregulating genes related to cholesterol synthesis, fatty acid, and gluconeogenesis. BYG also suppressed glucose transporters, adipogenic genes in the intestine and fat emulsification, showing potential as an anti-diabetic agent (Cao et al., 2017).
Cucurbitacin B
Cucurbitacin B (CuB), a prominent bioactive compound naturally occurring in cucumbers, exhibits significant anti-tumor activity, explicitly targeting prostate cancer cells, such as PC-3 and LNCaP, by inhibiting ACLY. The mode of action involves inducing apoptosis. This suggests that CuB has the potential to be explored as a promising agent in the development of treatment or preventive strategies against prostate cancer.
Preclinical studies
Previous studies have emphasised the remarkable capacity of CuB to selectively obstruct the growth of prostate cancer cells, including apoptosis with an IC50 value of approximately 0.3 µM for PC-3 and LNCaP cancer cells while exhibiting protective effects on normal prostate cells. In the preclinical mice model, CuB showcased significant chemo-preventive effects, reducing tumor formation and size by 79% without noticeable toxicity. Mechanistic analyses reveal that CuB explicitly targets and inhibits ACLY. Overexpression of ACLY counteracts CuB’s apoptotic effects, conforming ACLY as a direct CuB target. Overall, CuB demonstrates a strong chemo-preventive effect against prostate cancer through an anti-tumor mechanism involving ACLY inhibition (Gao et al., 2014).
Resveratrol
Resveratrol is a polyphenolic flavonoid widely used as a dietary supplement and is present in many different types of plants, mainly berry fruits. Its anti-inflammatory, antioxidant, and anticarcinogenic qualities are well established. Resveratrol may modulate ACLY expression, potentially inhibiting its activity.
Preclinical studies
Based on research, resveratrol administered for eight weeks after a 12-week high-fat diet significantly prevented dyslipidemia and hepatic steatosis in rats. Resveratrol reduced key ER stress markers and modulated gene expression, including ACLY, thereby curbing excessive fat accumulation in diet -induced obese rats (Pan et al., 2015).
Cinnamon polyphenol
Cinnamon polyphenol refers to a substance derived from cinnamon. Cinnamon is one of the oldest spices that have medicinal properties and shows antioxidant, antihyperlipidemic, anti-obesity and anti-inflammatory effects (Beejmohun et al., 2014; Gunawardena, Govindaraghavan, & Münch, 2014; Tuzcu et al., 2017).
Preclinical studies
Extract of cinnamon polyphenol significantly reduced the expression of hepatic transcription factors, such as LXR-α and SREBP-1c, in rats fed a high-fat diet (HFD). The extract also prominently suppressed hepatic ACLY expression and NF-KB p65 while enhancing PPAR-α, Nrf2, IRS-1, and HO-1 expressions. This suggests that cinnamon polyphenol lowers oxidative stress, hyperlipidemia, and inflammation in HFD rat livers (Tuzcu et al., 2017).
3-Thiadicarboxylic acid
3-Thiadicarboxylic acid is a sulfur-substituted fatty acid analogue known as 1,10 bis(carboxymethylthio)decane, exhibiting a hypolipidemic effect in normolipidemic rats.
Preclinical studies
3-Thiadicarboxylic acid treatment inhibits ACLY and fatty acid synthase, resulting in a significant reduction of cholesterol, FAs, and triglycerides, accompanied by a decrease in LDL cholesterol and VLDL triglycerides. These findings suggest a potential role for 3-thiadicarboxylic acid in modulating lipid metabolism, and it lowers hyperlipidemia (Al-Shurbaji et al., 1993; Skorve et al., 1993).
NDI-091143
NDI-091143 binds to a hydrophobic cavity close to the citrate site, inhibiting ACLY. With a ki of 7.0 ± 0.8 nM and IC50 values of 2.1 ± 0.3 nM (ADP-Glo assay) and 4.8 ± 0.05 nM (coupled enzyme assay), it competitively inhibits citrate substrate. ATP elicits non-competitive kinetics, whereas CoA induces mixed-type non-competitive kinetics. The compound disrupts citrate binding and recognition by inducing conformational changes in the citrate domain. This allosteric inhibition underscores the potential of the allosteric site as a promising target in drug discovery, enhancing the likelihood of ACLY inhibitors (Jha et al., 2021; Liang & Dai, 2022; Wang et al., 2022; Wei et al., 2019; Zhang et al., 2023).
A research study applying a “ring closing” strategy for imposition of structural constraints inspired by NDI-091143, a new macrocyclic compound known as compound 2, is a potent inhibitor of ACLY. Compound 2 showed similar binding affinity and ACLY inhibitory activity compared to NDI-091143, indicating that it could be a promising lead compound. Using the MDH coupled enzyme assay, NDI-091143 was the most effective inhibitor of ACLY with an IC50 of 44.0 ± 3.0 nM. Moreover, compound 2 demonstrated potent inhibitory activity against ACLY with an IC50 value of 69.7 ± 9.6 nM, like the effectiveness of NDI-091143, the measured IC50 values correlated with their anticipated binding free energy values, highlighting the importance of the “ring-closing” approach in achieving proficient ACLY inhibition (Zang et al., 2022).
Forrestiacids
Forrestiacids E-K (1-7), a group of distinctive triterpene-diterpene hybrids, have been isolated from Pseudotsuga forrestii. These compounds, identified through phytochemical and LC-MS/MS techniques, showcase a unique bicyclo [2.2.2] octene motif. Notably, forrestiacids J (6) and K (7) stand out as the first examples of [4+2]- type hybrids derived from a typical lanostane-type dienophile. Several compounds, especially forrestiacids J and K, exhibited notable inhibition of ACLY (IC50: 1.8 to 11µM). Docking studies confirmed strong binding affinities (-9.9 to -10.7 kcal/mol) with ACLY, emphasising their potential therapeutic significance in managing diseases related to ACLY (Zhou et al., 2023).
2-chloro-1, 3, 8-trihydroxy-6-methyl-9-anthrone
2-chloro-1, 3, 8-trihydroxy-6-methyl-9-anthrone is identified as a hypolipidemic agent with a primary assay IC50 of 283nM, and more specifically, a Ki of <100nM in opposition to the Mg citrate substrate. It is an active metabolite of microorganisms isolated from Penicillium sp., SC2193, a soil fungus, was examined. This culture produces numerous related anthraquinones and anthrones. There is evidence that 2-chloro-1, 3, 8-trihydroxy-6-methyl-9-anthrone is one of these anthrones and that it acts as a selective and competitive inhibitor of ACLY against Mg citrate as a substrate (Oleynek et al., 1995).
Antimycins
Antimycins, including antimycins A7 and A8, and well-known antimycins A1, A2, A3, and A4, were produced in the culture broth of a Streptomyces species. These compounds exhibit inhibitory activity against ACLY, with Ki values ranging from 4 to 60 nM against the substrate Mg citrate. Among the isolated compounds, Antimycins A2 and A8 emerged as the highly effective inhibitors of ACLY, displaying Ki values of 4.2 µM and 4.0 µM, respectively (Barrow et al., 1997).
10, 11-dehydrocurvularin (DCV
10, 11-dehydrocurvularin (DCV) is an organic macrolide derived from fungi that has demonstrated remarkable therapeutic potential as a potent anti-cancer, anti-infective, and immune-modulatory agent. By using chemo proteomic profiling, it was found that the DCV is a potent irreversible ACLY inhibitor. This uncovers the mechanism of action of DCV and its analogous against cancer (Deng et al., 2019).
Furan carboxylic derivatives
Furan carboxylic derivatives were systematically analysed for their inhibitory potential against ACLY. Among 1947 furan carboxylic derivatives evaluated through dual docking algorithms, four subtypes of (benzo) furan carboxylic acids emerged as potent ACLY inhibitors. From the top-ranked 24 virtual hits, 11 compounds were validated in vitro, with lead compound A1 displaying a notable IC50 of 4.1 µM, with A3 and C4 showing IC50 values of 11.9 and 13.8 µM, respectively. This study enhances our understanding of furan carboxylic derivatives as ACLY inhibitors, revealing promising candidates for further optimisation in anticancer drug development (Jernigan et al., 2017).
Figure 5
Chronological progression and significant achievements in the research on ACLY from its initial discovery to ongoing clinical trials .
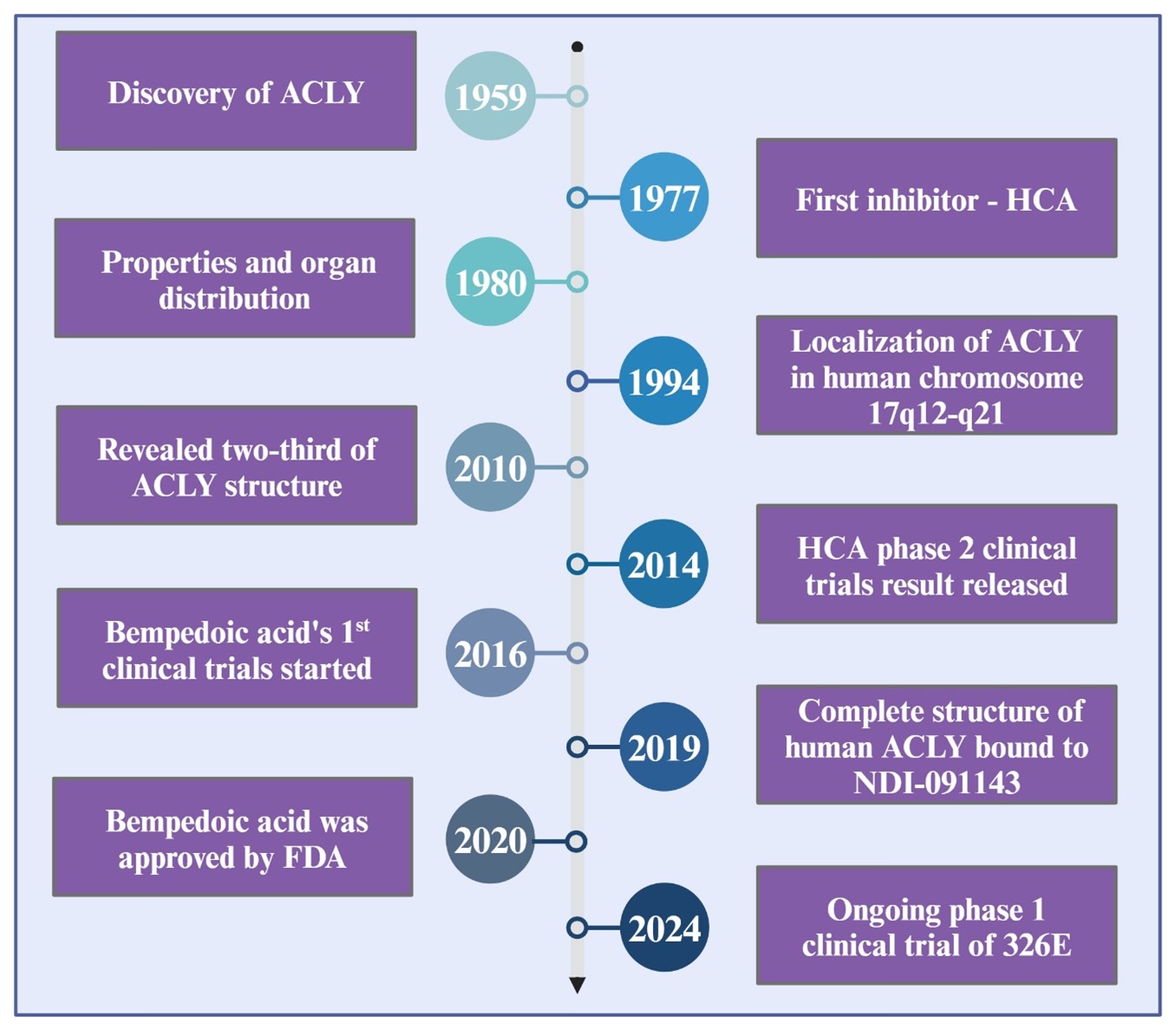
Table 2
Contemporary pharmaceutical approaches aimed at inhibiting ACLY.
Candidate | Type | Advantages | Administration | Disease | Development status | Sponsor/Reference |
---|---|---|---|---|---|---|
(-)-hydroxycitric acid | Natural compound | Reduce food intake, lowers weight, reduce oxidative stress, increase satiety | Orally | Obesity, oxidative stress. | NCT01238887, Phase 2, completed | University of Florida; (Sharma et al., 2018) Clinical Trails, 2024 |
Bempedoic acid | Small molecule | Lowers LDL | Orally | HeFH, ASCVD | Approved (USA and European Union, 2020) | Esperion Therapeutics, Inc; FDA, 2024 |
326E | Small molecule | Increase cholesterol efflux and reduce hepatic lipogenesis. | Orally | Hypercholesterolemia | Preclinical (hamster and rhesus monkey); Ongoing phase 1 clinical trial | Burgeon Therapeutics; (Xie et al., 2023). |
Curcumin | Natural compound | Increase HDL-C, reduce VLDL-C, triglycerides | Orally | NAFLD, hyperlipidemia | Preclinical (mice, broiler chickens, Wistar rat) | |
SB-204990 | - | Prevent metabolic issues linked to poor diet and aging | Orally | Cancer | Preclinical (mice) | (Chen et al., 2023; Sola-García, Cáliz-Molina, Espadas, & Petr, 2023) |
BMS-303141 | - | Protect against renal injuries. | Orally | HCC | Preclinical (mouse, mice) | (Zheng et al., 2021); (Zhan et al., 2022); (Guo et al., 2020) |
2-Furoic acid |
| elevated HDL receptor activity and decreased LDL receptor activity | Orally | Acts in small intestine and liver | Preclinical (rat) | |
MEDICA 16 | Free fatty acid 1 | Exhibit antidiabetogenic and hypolipidemic properties | Orally | Antilipemic drug | Preclinical (mice, rat) | |
Baker’s yeast glucan (BYG) | Polysaccharide | Reduce hepatic disorder and blood glucose | Orally | Anti-diabetic drug | Preclinical (mice) | |
Cucurbitacin B | Natural compound | Induce apoptosis | Oral gavage | Cancer | Preclinical (mice) | |
Resveratrol | Natural compound | Protect against ER stress and hepatic steatosis | Orally | Hepatic steatosis | Preclinical (rat) | |
Cinnamon polyphenol | Natural compound | suppresses hepatic ACLY expression, lowers hyperlipidemia | Orally | Liver, hyperlipidemia | Preclinical (rat) | |
3-Thiadicarboxylic acid |
| Lowers hyperlipidemia and modulate lipid metabolism | Gastric intubation | hyperlipidemia | Preclinical (rat) | |
NDI-091143 | - | Revealed that the allosteric site as a promising target | - | - | Enzymatic assay & In silico analyses | |
Forrestiacids J & K | Natural compound | exhibited notable inhibition of ACLY | - | - | Enzymatic assay & In silico analyses | |
2-chloro-1, 3, 8-trihydroxy-6-methyl-9-anthrone | Natural compound | opposition to the Mg citrate substrate and lowers hyperlipidemia | - | hyperlipidemia | Enzymatic assay | |
Antimycins | - | inhibitory activity against ACLY’s Mg citrate substrate | - | - | Enzymatic assay | |
10, 11-dehydrocurvularin | organic macrolide | potent irreversible ACLY inhibitor | - | Cancer | Enzymatic assay | |
Furan carboxylic derivatives | - | Potential inhibitor of ACLY | - | Cancer | Enzymatic assay & In silico analyses | |
Radicicol | macrolide | primarily targets Hsp90, blocks insulin secretion | - | - | Enzymatic assay | |
(+)-2,2-difluorocitrate | - | Regulate acetyl-CoA synthesis | - | - | Enzymatic assay | |
Crocins I ana II | Natural compound | Potentially inhibits ACLY | - | - | Enzymatic assay | |
Purpurone | non-crystalline glassy solid | Impedes FA synthesis | - | - | Enzymatic assay | |
Alkenyl diacids | - | Anti-tumor effect | - | - | Enzymatic assay | |
Formyl phloroglucinol meroterpenoids | - | suppression of hepatocyte lipogenesis | - | - | Enzymatic assay |
Radicicol
Radicicol, a macrolide initially isolated from Monosporium bonoredn, was identified as an ACLY inhibitor. It exhibited non-competitive inhibition of ACLY with Ki values of 13 µM for citrate and 7 µM for ATP. Radicicol, known for its primary target Hsp90, also blocks insulin secretion in pancreatic-β cells stimulated by glucose, highlighting its diverse biological activities (Granchi, 2018; Ki et al., 2000).
4.21. (+)-2,2-difluorocitrate
(+)-2,2-difluorocitrate and (-)-2,2-difluorocitrate is enantiomers act as a potent ACLY inhibitors with Kis = 0.7 µM and Kis = 15 µM respectively, with the (+)-enantiomer exhibiting greater affinity. Only the (+)-enantiomer undergoes chemical processing. In aconitase reactions, (-)-2,2-difluorocitrate competes with citrate and irreversibly inactivates aconitase, while the inhibitor potency of (+)-enantiomer is relatively low. These findings suggest that the (+)-enantiomer can specifically regulate acetyl-CoA synthesis in vivo without interfering with the citric acid cycle (Saxty et al., 1991).
Crocins
Crocins are extracted from Gardenia jasminoides, utilised as seasonings and food colouring, and have medicinal benefits for human health. The fruit G. jasminoides is the source of crocins I and II, which have been demonstrated to inhibit ACLY. The IC50 value for Crocin I is 36.3 ± 6.24, and for Crocin II is 29.7 ± 7.41 µM (Guan et al., 2021).
Purpurone
Purpurone is purple in colour and a non-crystalline glassy solid with antioxidant activities. It was isolated from the sponge Lotrochota sp, with an IC50 value of 7 µM. Purpurone inhibits ACLY in a dose-responsive way. It hinders the synthesis of FAs without impacting the synthesis of cholesterol. At 100 µg/ml, there is no effect on the viability of HepG2 cells or cellular ATP levels. The discovery of novel derivative products of purpurone as an ACLY inhibiting agent has been assisted by introducing a new rapid synthesis method for purpurone ( ; Chan et al., 1993) (Granchi, 2018).
Alkenyl diacids
Alkenyl diacids can inhibit ACLY directly, and this potent inhibitor has been found in several brand-new long chains. Compound 18f showed an IC50 value of 1.5 µmol/L and proved to be the most potent inhibitor of ACLY. Novel compounds such as 25f have been generated through ester formation from 18f. The resulting compounds demonstrated increased antitumor cell proliferation effects while maintaining their inhibitory activity on ACLY (Song et al., 2022).
Formyl phloroglucinol meroterpenoids
Formyl phloroglucinol meroterpenoids consisting of eumaidials A-C (1-3) have been isolated from the leaves of Eucalyptus globulus subsp. Maidenii. The 1st identified β-caryophyllene-based formyl phloroglucinol meroterpenoid from Eucalyptus is Eumaidial A (1). A total of 10 known analogues in compounds 2 and 3 revealed suppression of hepatocyte lipogenesis, while compounds 1-4 and 10 showed inhibitory activity towards ACLY (Hu et al., 2024).
The overall chronological progression and significant achievements in the research on ACLY from its initial discovery to ongoing clinical trials are shown in Figure 5.
Challenges and future perspective
Developing ACLY inhibitors with high selectivity and specificity is a significant hurdle. The key to minimising off-target effects is attaining targeted inhibition without compromising related enzymes or essential cellular processes. ACLY has several roles in different tissues, including white adipose tissue, liver, brain, heart muscles, pancreatic cells, and small intestines (Chypre et al., 2012). Therefore, developing inhibitors that can only target ACLY in specific tissues or cell types while sparing others poses a severe obstacle.
Biomarker discovery involves thoroughly examining molecular, imaging, or clinical data to identify indicators linked to ACLY dysregulation and inhibitor response. Applying a personalised approach based on these biomarkers might increase the efficacy and accuracy of ACLY-targeted interventions, which may result in improved patient outcomes and individualised treatment plans. Continued research into the structural details of ACLY can yield the necessary knowledge for designing more effective and targeted inhibitors. High-resolution structural data can direct inhibitor development with increased potency. Investigating the non-metabolic roles of ACLY and its connection to signalling pathways might lead to the discovery of novel therapeutic opportunities. Understanding the broader biological context of ACLY may increase its potential uses. The biomarker discovery, precision medicine, understanding of non-metabolic functions and structural insights will influence the direction of ACLY research and therapeutics in future. An overall summary of the contemporary pharmaceutical approaches aimed at inhibiting ACLY is given in Table 2.
Conclusion
ATP Citrate Lyase (ACLY) has emerged as a pivotal enzyme at the crossroads of metabolic and epigenetic regulation, significantly influencing lipid biosynthesis and gene expression through histone acetylation. This review has elucidated ACLY's central role in cellular metabolism, providing deep insights into its structure, function, and the regulatory mechanisms that govern its activity in various tissues. ACLY's involvement in critical metabolic pathways highlights its importance in health and disease. It is notably overexpressed in several cancers and metabolic disorders, supporting the biosynthetic demands of rapidly proliferating cells and contributing to metabolic dysfunction. The enzyme's role in producing acetyl-CoA, a key metabolic and epigenetic regulator, underscores its potential as a therapeutic target, particularly in metabolic syndromes, cardiovascular diseases, and oncology.
This review navigates the complex field of ACLY, shedding light on its significant role in cellular metabolism and its influence on various diseases, from its basic biochemistry and molecular biology to the creation of ACLY inhibitors and their translation into preclinical and clinical study, evaluating the therapeutic potential of therapies targeting ACLY. Concluding our analyses, the review clarifies the difficulties observed in developing ACLY inhibitors and discusses possible methods for overcoming these challenges. Finally, the direction into the future reveals promising avenues for more study and advancement in the dynamic and developing area of ACLY modulation. Overall, this review provides a holistic grasp of ACLY and its possible therapeutic implications, providing a foundation for future research and innovation.
The review also details the development and mechanical action of various ACLY inhibitors, which have shown promise in preclinical and clinical settings. Notably, inhibitors like bempedoic acid have reached clinical use, offering new therapeutic avenues for managing conditions like hypercholesterolemia and cardiovascular disease. The discussion of ACLY inhibitors extends to emerging compounds in clinical trials, reflecting a robust pipeline of potential therapies to modulate this enzyme's activity. Despite significant advances, the development of ACLY inhibitors faces challenges, particularly in achieving selectivity and minimising off-target effects. The tissue-specific roles of ACLY suggest that future inhibitors must be finely tuned to target specific cellular contexts without disrupting the enzyme's essential functions in non-target tissues. The review advocates for a continued focus on unravelling the complex regulation of ACLY and its broader biological roles. Such an understanding could unveil novel therapeutic strategies that leverage ACLY's central position in metabolic and epigenetic networks. Moreover, integrating ACLY modulation with other therapeutic approaches could enhance the efficacy and precision of treatments for ACLY-associated diseases.
In conclusion, ATP Citrate Lyase remains a promising target for therapeutic intervention. Continued research and development driven by the insights outlined in this review may lead to innovative treatments that better manage metabolic disorders, cancer, and other conditions associated with dysregulated ACLY activity. This endeavor will require a multidisciplinary approach, integrating detailed biochemical studies, advanced drug design, and clinical trials to fully harness ACLY inhibitors' therapeutic potential.
Conflicts of interest
Given his role as Editor in Chief, Kannan RR Rengasamy has not been involved the peer review of this article. Full responsibility for the editorial process for this article was delegated to Co-Editor Carlos L. Cespedes Acuña. All authors declare that there is no conflict of interest associated with this manuscript.
Author contributions
Conceptualization: Kannan RR Rengasamy; Writing Original Draft: Aswathi KN Biju; Literature collection and image, drafting the content of this review: Kannan RR Rengasamy, Rahul Raj CL, Review and editing: Kannan RR Rengasamy, Final Approval and Submission: Kannan RR Rengasamy.
Abbreviations
ACLY: ATP citrate lyase
ATP: Adenosine triphosphate
ADP: Adenosine diphosphate
Pi: Inorganic phosphate
LDLr: Low-density lipoprotein receptor
CCL: Citrullin-CoA lyase
CCS: Citrulline CoA synthase
CS: Citrate synthase
OAA: Oxaloacetate
PDC: Pyruvate dehydrogenase complex
cAMP: Cyclic adenosine monophosphate
DNA: Deoxyribonucleic acid
MCF-7: Michigan cancer foundation-7
TCA: Tricarboxylic acid
SREBP-1α: Sterol regulatory element binding protein1α
SRE: Sterol response element
AMPK: AMP-activated protein kinase
FAs: Fatty acids
DNL: De novo lipogenesis
ACC: Acetyl-CoA carboxylase
FASN: Fatty acid synthase
SCD: Stearoyl-CoA desaturase
HMGCR: 3-hydroxy-3-methylglutaryl coenzyme A reductase
FPP: Farnesyl pyrophosphate
GG-PP: Geranylgeranyl pyrophosphate
ASCVD: Atherosclerotic cardiovascular disease
LDL-C: Low-density lipoprotein-cholesterol
ABCG5/8: ATP-binding cassette transporter G5/8
NAFLD: Non-alcoholic fatty liver disease
AD: Alzheimer’s disease
FD: Familial dysautonomia
HCA: Hydroxycitric acid
TAM: Tamoxifen
ACC-α: Acetyl CoA carboxylase alpha
HDL-C: High-density lipoprotein-cholesterol
hsCPR: High-sensitivity C-reactive protein
HeFH: Heterozygous familial hyper-cholesterolemia
OAT: Organic anion transporter
BMMs: Bone Marrow-Derived Macrophages
HFHC: High-fat high-cholesterol
OPA: Oleic and palmitic acid
HCC: Hepatocellular carcinoma
HFD: High-fat diet
DCV: Dehydrocurvularin
BYG: Baker’s yeast glucan